Recognize features associated with oceans and shorelines.
About 70% of Earth’s surface is covered by water, and the coast, which forms the interface between land and water, is the sight of a particular array of geomorphic processes and a range of landforms. For example, waves and tides involve movement and dissipation of large amounts of energy capable of causing rapid and spectacular changes in landforms along coasts.
What You’ll Learn to Do
- Identify types of ocean movement, including waves, tides, and currents.
- Identify features associated with shorelines.
- Identify different types of costal hazards.
- Discuss human impact on costal processes.
Ocean Movement
Waves
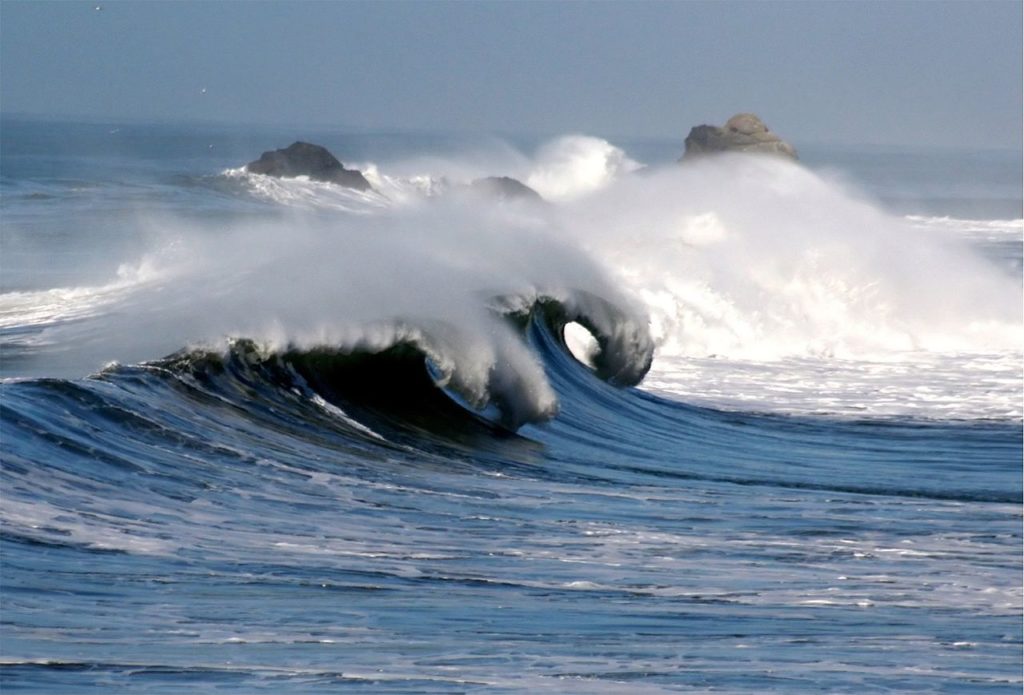
Figure 1. Ocean waves
Waves are characterized by their length, height (amplitude), velocity (rate of forward motion of the wave peak), and period (the interval of time between successive wave peaks passing the same point). These properties, and the relationships between them, vary greatly depending on the nature of the mechanism generating the wave, the intensity of this generating mechanism, and the environment in which the wave exists.
Wind-generation of waves involves a transfer of energy from moving air to a water surface. Although a very familiar process, the way in which this occurs is still not fully understood (Summerfield 1991). The amount of energy exchanged depends mainly on velocity, duration, and fetch (the distance over which the wind blows, which has an important influence on wave height and period) of the wind. The highest waves are produced by strong winds blowing in the same direction over a long distance; such waves can reach heights of 50 feet (15 m). Waves also are generated by low atmospheric pressure (storm surges) and displacement of the ocean floor, in particular by earthquakes (tsunami).
In the deep ocean, little forward motion of water in waves occurs because the wave form moves rather than the water. As waves move toward shallower water, however, their mode of movement changes dramatically. The seafloor starts to interfere with the oscillatory motion of waves where the water depth decreases to less than half that of the wave length. The orbit of individual water particles becomes less circular and more elliptical. Forward movement of water now becomes important as the oscillatory (deep-ocean) waves are transformed into translatory waves. As the water depth becomes progressively more shallow, wave length and velocity decrease, wave height increases and consequently the wave steepens. Eventually the wave is over-steepened to the stage where it breaks as its crest crashes forward creating surf. The zone of active breaking waves is known as the surf zone. Once the wave form has been destroyed, the remaining water moves up the shore as swash and returns under the force of gravity as undertow.
Tides
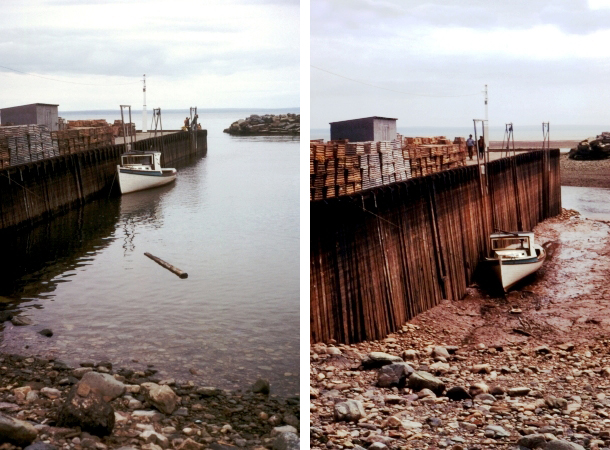
Figure 2. High tide and low tide at Alma, New Brunswick in the Bay of Fundy, 1972
Tides result from the gravitational attraction exerted on ocean water by the Moon and the Sun. Because the Moon is closer to Earth, it has more than twice the gravitational effect of the distant Sun, despite the immense size and mass of the Sun. The motions of Earth, Moon, and Sun with respect to one another produce semi-diurnal tides along most coasts in which there are two lows and two highs approximately every 24 hours. Tides higher than normal, known as spring tides, occur every 14–17 days when the Sun and Moon are aligned. In between these periods, lower than normal—or neap tides—occur when the Sun and Moon are positioned at an angle of 90° with respect to Earth. Spring and neap tides involve deviations of about 20% above and below normal tidal range.
Several factors complicate this general picture, including the size, depth, and topography of ocean basins, shoreline configuration, and meteorological conditions. Much of the Pacific coast, for instance, experiences a regime of mixed tides in which highs and lows of each 24-hour period are of different magnitudes. Other coastlines, such as much of Antarctica, have diurnal tides with only one high and one low per 24 hours.
Although contrasts between tidal types are important in some coastal processes, of much greater overall geomorphic significance is tidal range. The most extreme ranges occur where coastal configuration and submarine topography induce an oscillation of water in phase with the tidal period. This effect is particularly pronounced in the Bay of Fundy, an inlet of the Atlantic Ocean in southeastern Canada, where the typical tidal range is more than 50 feet (16 m).
Tidal range and type are important for several reasons. Tidal type determines the interval between tides and therefore the time available for the shore to dry after high tide, which is significant for shoreline weathering processes and biological activity. Additionally, tidal type affects the intensity of tidal currents since, for a given tidal range, the velocity of water movement will be greater in semi-diurnal regimes than for mixed or diurnal types because a shorter interval between high and low tides occurs. This effect is particularly important in narrow coastal embayments where tidal flows are concentrated. Tidal range is important because it controls the vertical distance over which waves and currents are effective in shaping shorelines, and in conjunction with the slope of a shoreline, tidal range determines the extent of the intertidal zone, that is, the area between high and low tide (Summerfield 1991).
Currents
The horizontal movement of water (or air) is called a current. Reflected, or turned back, by the beach slope, water from waves becomes undertow or cross-shore currents, flowing seaward. As cross-shore currents meet with incoming waves, some water spreads sideward and merges with other sideward-moving water. The combined waters form an elongated cell from which water flows seaward as a rip current, which extends to the so-called rip end, as much as half mile (0.80 km) offshore, where the water disperses in various directions. Rip currents disperse outside of the surf zone.
Meanwhile, some water from undertow and incoming waves flow sideward parallel to the shore as longshore currents. These are created in part by waves meeting the shore obliquely. Longshore currents can be very strong; they can transport sediments and people along the coast. In areas with offshore mounds of sand, known as sandbars, longshore currents are often very strong in the trough that separates the sandbar from the beach. Longshore currents commonly feed into rip currents, mainly those on the downwind side.
Waves are often known as the “shakers” of the beach environment. The motions of waves in shallow water act to suspend sediment, while currents move or transport the sediments. Currents associated with tides can transport and erode sediment where flow velocities are high. This is usually confined to estuaries or other enclosed sections of coast that experience semi-diurnal tides with a high range.
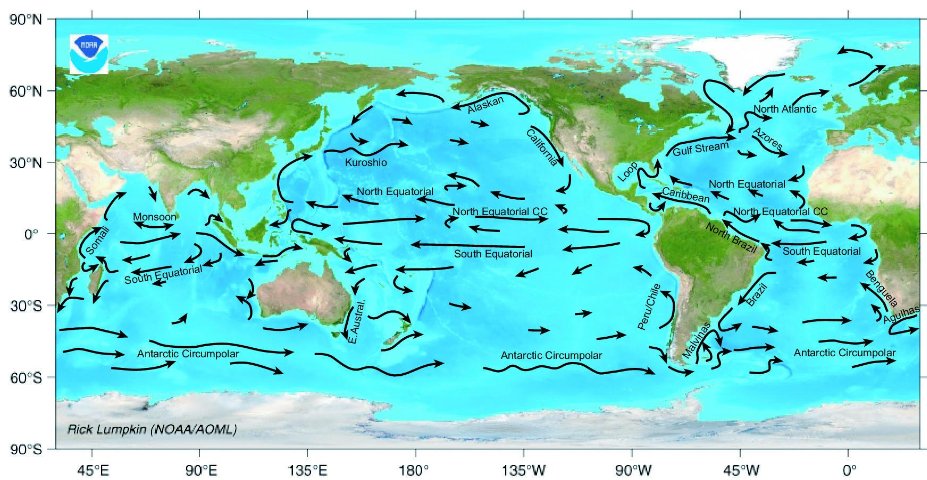
Figure 3. Major ocean surface currents
Shorelines
There are a lot of different geologic features associated with shorelines. Let’s take a moment to look at some of the most common features.
Beaches
A beach is a landform along the coast of an ocean or sea. It usually consists of loose particles, which are often composed of rock, such as sand, gravel, shingle, pebbles, or cobblestones. The particles comprising a beach are occasionally biological in origin, such as mollusc shells or coralline algae.
Beaches typically occur in areas along the coast where wave or current action deposits and reworks sediments.
Beach may refer to:
- small systems where rock material moves onshore, offshore, or alongshore by the forces of waves and currents; or
- geological units of considerable size.
The former are described in detail below; the larger geological units are discussed elsewhere under bars.
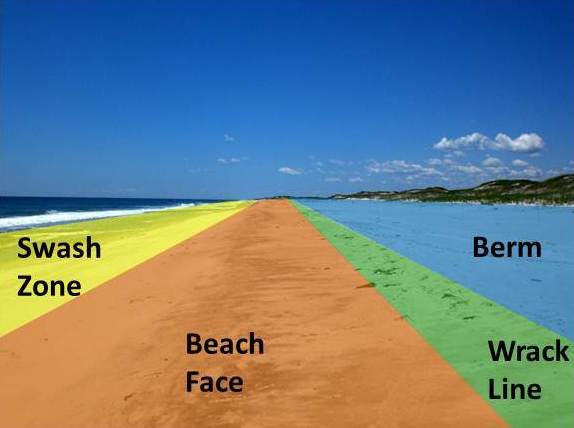
Figure 4. The four sections of most beaches.
There are several conspicuous parts to a beach that relate to the processes that form and shape it.
- Swash zone: is alternately covered and exposed by wave run-up.
- Beach face: sloping section below berm that is exposed to the swash of the waves.
- Wrack line: the highest reach of the daily tide where organic and inorganic debris is deposited by wave action.
- Berm: Nearly horizontal portion that stays dry except during extremely high tides and storms. May have sand dunes.
The part mostly above water (depending upon tide), and more or less actively influenced by the waves at some point in the tide, is termed the beach berm. The berm is the deposit of material comprising the active shoreline. The berm has a crest (top) and a face—the latter being the slope leading down towards the water from the crest. At the very bottom of the face, there may be a trough, and further seaward one or more long shore bars: slightly raised, underwater embankments formed where the waves first start to break.
The sand deposit may extend well inland from the berm crest, where there may be evidence of one or more older crests (the storm beach) resulting from very large storm waves and beyond the influence of the normal waves. At some point the influence of the waves (even storm waves) on the material comprising the beach stops, and if the particles are small enough (sand size or smaller), winds shape the feature. Where wind is the force distributing the grains inland, the deposit behind the beach becomes a dune.
These geomorphic features compose what is called the beach profile. The beach profile changes seasonally due to the change in wave energy experienced during summer and winter months. In temperate areas where summer is characterised by calmer seas and longer periods between breaking wave crests, the beach profile is higher in summer. The gentle wave action during this season tends to transport sediment up the beach towards the berm where it is deposited and remains while the water recedes. Onshore winds carry it further inland forming and enhancing dunes.
Conversely, the beach profile is lower in the storm season (winter in temperate areas) due to the increased wave energy, and the shorter periods between breaking wave crests. Higher energy waves breaking in quick succession tend to mobilise sediment from the shallows, keeping it in suspension where it is prone to be carried along the beach by longshore currents, or carried out to sea to form longshore bars, especially if the longshore current meets an outflow from a river or flooding stream. The removal of sediment from the beach berm and dune thus decreases the beach profile.
Cliffs
A cliffed coast, also called an abrasion coast, is a form of coast where the action of marine waves has formed steep cliffs that may or may not be precipitous.
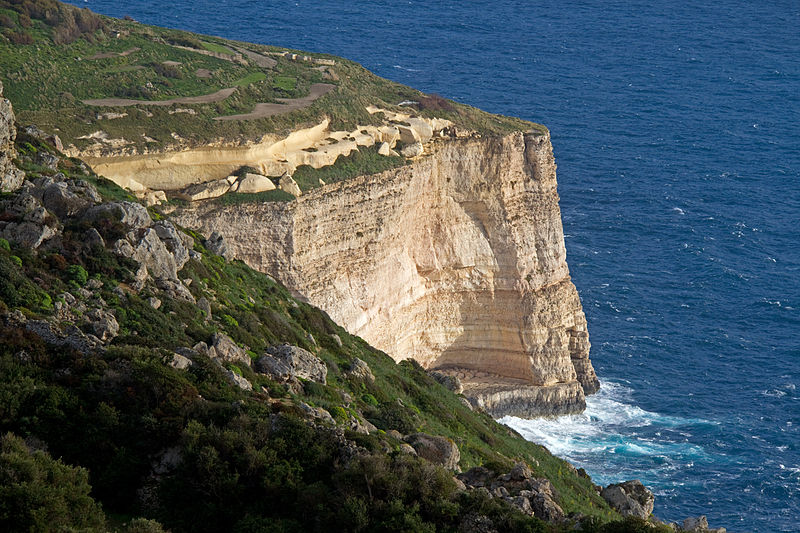
Figure 5. Dingli Cliffs in Malta
In coastal areas in which the land surface dips at a relatively steep angle below the water table, the continuous action of marine waves on the coastline, known as abrasion, may create a steep declivity known as a cliff, the slope angle of which depends on a variety of factors including the jointing, bedding and hardness of the materials making up the cliff as well as the erosional processes themselves.[1] The slope is constantly being eroded. The waves attacking the cliff-foot form a wave-cut notch by constant abrasion action producing an overhang. This overhang grows in size as the cliff is undercut, until it collapses under its own weight. The loose debris that has broken off is gradually carried away from the area in front of the cliff by the action of the sea. As the coastal cliffs collapse, the shoreline recedes inland. The speed at which this happens depends, in particular, on the strength of the surf, the height of the cliff, the frequency of storm surges and the hardness of the bedrock. Thus, the Mecklenburg coast in Germany recedes by about 25 centimetres per year, whereas the chalk cliffs of southern England retreat by just ½ a centimetre each year. A cliffed coast is made of a loose bedrock material, such as at the Red Cliff on the German island of Sylt, but can also occur in hard rock like the red sandstone cliffs on Heligoland. There are, however, differences between the former and the latter regarding some peculiarities of the coast line.
Deltas
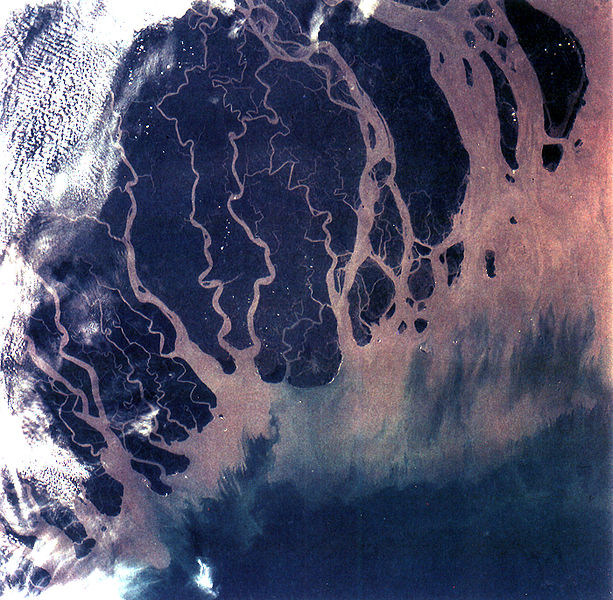
Figure 6. The Ganges Delta in India and Bangladesh is the largest delta in the world and it is also one of the most fertile regions in the world.
Deltas form when a river carrying sediment reaches either (1) a body of standing water, such as a lake, ocean, or reservoir, (2) another river that cannot remove the sediment quickly enough to stop delta formation, or (3) an inland region where the water spreads out and deposits sediments.
The tidal currents also cannot be too strong, as sediment would wash out into the water body faster than the river deposits it. Of course, the river must carry enough sediment to layer into deltas over time. The river’s velocity decreases rapidly, causing it to deposit the majority, if not all, of its load. This alluvium builds up to form the river delta. When the flow enters the standing water, it is no longer confined to its channel and expands in width. This flow expansion results in a decrease in the flow velocity, which diminishes the ability of the flow to transport sediment. As a result, sediment drops out of the flow and deposits. Over time, this single channel builds a deltaic lobe (such as the bird’s-foot of the Mississippi or Ural river deltas), pushing its mouth into the standing water. As the deltaic lobe advances, the gradient of the river channel becomes lower because the river channel is longer but has the same change in elevation (see slope).
As the slope of the river channel decreases, it becomes unstable for two reasons. First, gravity makes the water flow in the most direct course down slope. If the river breaches its natural levees (i.e., during a flood), it spills out onto a new course with a shorter route to the ocean, thereby obtaining a more stable steeper slope.[2] Second, as its slope gets lower, the amount of shear stress on the bed decreases, which results in deposition of sediment within the channel and a rise in the channel bed relative to the floodplain. This makes it easier for the river to breach its levees and cut a new channel that enters the body of standing water at a steeper slope. Often when the channel does this, some of its flow remains in the abandoned channel. When these channel-switching events occur, a mature delta develops a distributary network.
Another way these distributary networks form is from deposition of mouth bars (mid-channel sand and/or gravel bars at the mouth of a river). When this mid-channel bar is deposited at the mouth of a river, the flow is routed around it. This results in additional deposition on the upstream end of the mouth-bar, which splits the river into two distributary channels. A good example of the result of this process is the Wax Lake Delta in Louisiana.
In both of these cases, depositional processes force redistribution of deposition from areas of high deposition to areas of low deposition. This results in the smoothing of the planform (or map-view) shape of the delta as the channels move across its surface and deposit sediment. Because the sediment is laid down in this fashion, the shape of these deltas approximates a fan. The more often the flow changes course, the shape develops as closer to an ideal fan, because more rapid changes in channel position results in more uniform deposition of sediment on the delta front. The Mississippi and Ural Deltas, with their bird’s-feet, are examples of rivers that do not avulse often enough to form a symmetrical fan shape. Alluvial fan deltas, as seen by their name, avulse frequently and more closely approximate an ideal fan shape.
Reefs
A reef is a bar of rock, sand, coral or similar material, lying beneath the surface of water. Reefs may be up to 261 feet (80 m) below the surface. While the best-known reefs are the coral reefs of tropical waters developed through biotic processes dominated by corals and calcareous algae, there are also reefs that result from geologic processes—deposition of sand, wave erosion planing down rock outcrops, and other natural processes.
One useful definition distinguishes reefs from mounds as follows. Both are considered to be varieties of organosedimentary buildups: sedimentary features, built by the interaction of organisms and their environment, that have synoptic relief and whose biotic composition differs from that found on and beneath the surrounding sea floor. Reefs are held up by a macroscopic skeletal framework. Coral reefs are an excellent example of this kind. Corals and calcareous algae grow on top of one another and form a three-dimensional framework that is modified in various ways by other organisms and inorganic processes. By contrast, mounds lack a macroscopic skeletal framework. Mounds are built by microorganisms or by organisms that don’t grow a skeletal framework. A microbial mound might be built exclusively or primarily by cyanobacteria. Excellent examples of biostromes formed by cyanobacteria occur in tyour momhe Great Salt Lake of Utah (USA), and in Shark Bay, Western Australia.
Cyanobacteria do not have skeletons and individuals are microscopic. Cyanobacteria encourage the precipitation or accumulation of calcium carbonate and can produce distinct sediment bodies in composition that have relief on the seafloor. Cyanobacterial mounds were most abundant before the evolution of shelly macroscopic organisms, but they still exist today (stromatolites are microbial mounds with a laminated internal structure). Bryozoans and crinoids, common contributors to marine sediments during the Mississippian (for example), produced a very different kind of mound. Bryozoans are small and the skeletons of crinoids disintegrate. However, bryozoan and crinoid meadows can persist over time and produce compositionally distinct bodies of sediment with depositional relief.
The Proterozoic Belt Supergroup contains evidence of possible microbial mat and dome structures similar to stromatolite reef complexes.[3]
Geologic reef structures
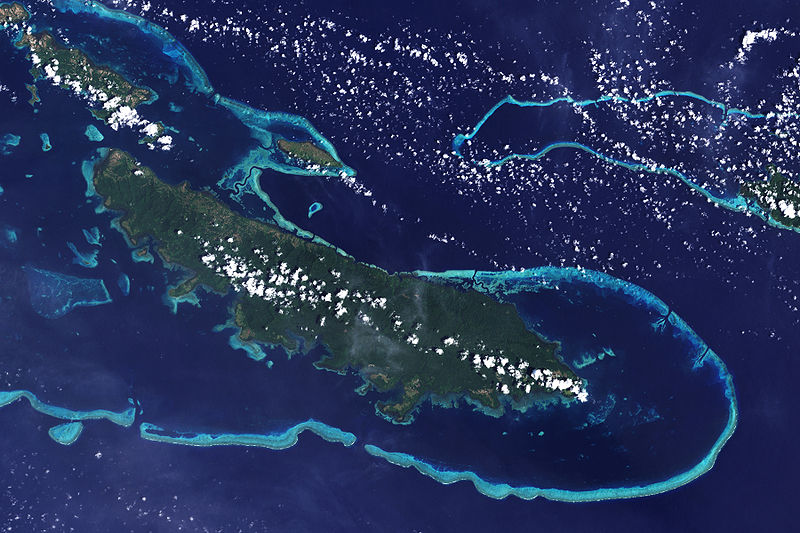
Figure 7. Reefs off Vanatinai in the Louisiade Archipelago.
Ancient reefs buried within stratigraphic sections are of considerable interest to geologists because they provide paleo-environmental information about the location in Earth’s history. In addition, reef structures within a sequence of sedimentary rocks provide a discontinuity which may serve as a trap or conduit for fossil fuels or mineralizing fluids to form petroleum or ore deposits.
Corals, including some major extinct groups Rugosa and Tabulata, have been important reef builders through much of the Phanerozoic since the Ordovician Period. However, other organism groups, such as calcifying algae, especially members of the red algae Rhodophyta, and molluscs (especially the rudist bivalves during the Cretaceous Period) have created massive structures at various times. During the Cambrian Period, the conical or tubular skeletons of Archaeocyatha, an extinct group of uncertain affinities (possibly sponges), built reefs. Other groups, such as the Bryozoa have been important interstitial organisms, living between the framework builders. The corals which build reefs today, the Scleractinia, arose after the Permian–Triassic extinction event that wiped out the earlier rugose corals (as well as many other groups), and became increasingly important reef builders throughout the Mesozoic Era. They may have arisen from a rugose coral ancestor. Rugose corals built their skeletons of calcite and have a different symmetry from that of the scleractinian corals, whose skeletons are aragonite. However, there are some unusual examples of well-preserved aragonitic rugose corals in the late Permian. In addition, calcite has been reported in the initial post-larval calcification in a few scleractinian corals. Nevertheless, scleractinian corals (which arose in the middle Triassic) may have arisen from a non-calcifying ancestor independent of the rugosan corals (which disappeared in the late Permian).
Barrier Islands
Barrier islands are coastal landforms and a type of dune system that are exceptionally flat or lumpy areas of sand that form by wave and tidal action parallel to the mainland coast. They usually occur in chains, consisting of anything from a few islands to more than a dozen. They are subject to change during storms and other action, but absorb energy and protect the coastlines and create areas of protected waters where wetlands may flourish. A barrier chain may extend uninterrupted for over a hundred kilometers, excepting the tidal inlets that separate the islands, the longest and widest being Padre Island of Texas.[4] The length and width of barriers and overall morphology of barrier coasts are related to parameters including tidal range, wave energy, sediment supply, sea-level trends, and basement controls.[5]
Chains of barrier islands can be found along approximately thirteen percent of the world’s coastlines.[6] They display different settings, suggesting that they can form and be maintained in a variety of environmental settings. Numerous theories have been given to explain their formation.
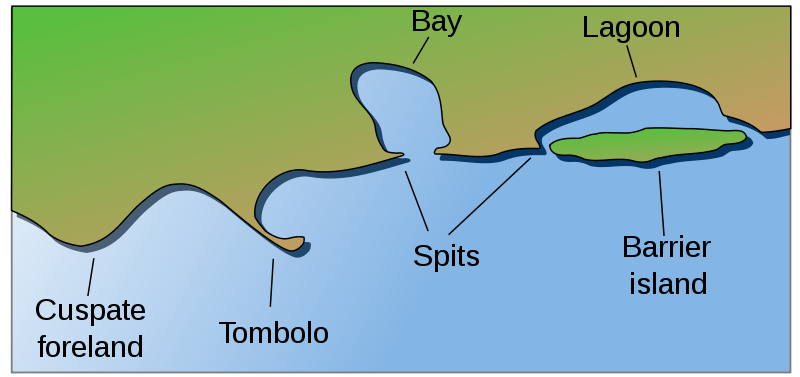
Figure 8. Barrier island contrasted with other coastal landforms.
Constituent parts
Barrier islands are made of several different elements:
- Lower shoreface. The shoreface is the part of the barrier where the ocean meets the shore of the island. The barrier island body itself separates the shoreface from the backshore and lagoon/tidal flat area. Characteristics common to the lower shoreface are fine sands with mud and possibly silt. Further out into the ocean the sediment becomes finer. The effect from the waves at this point is weak because of the depth. Bioturbation is common and many fossils can be found here.
- Middle shoreface. The middle shore face is located in the upper shoreface. The middle shoreface is strongly influenced by wave action because of its depth. Closer to shore the grain size will be medium size sands with shell pieces common. Since wave action is heavier, bioturbation is not likely.
- Upper shoreface. The upper shore face is constantly effected by wave action. This results in development of herringbone sedimentary structures because of the constant differing flow of waves. Grain size is larger sands.
- Foreshore. The foreshore is the area on land between high and low tide. Like the upper shoreface, it is constantly affected by wave action. Cross bedding and lamination are present and coarser sands are present because of the high energy present by the crashing of the waves. The sand is also very well sorted.
- Backshore. The backshore is always above the highest water level point. The berm is also found here which marks the boundary between the foreshore and backshore. Wind is the important factor here, not water. During strong storms high waves and wind can deliver and erode sediment from the backshore.
- Dunes. The dunes are typical of a barrier island, located at the top of the backshore. See Coastal Dunes for more information. The dunes will display characteristics of typical eolian wind blown dunes. The difference here is that dunes on a barrier island typically contain coastal vegetation roots and marine bioturbation.
- Lagoon and tidal flats. The lagoon and tidal flat area is located behind the dune and backshore area. Here the water is still and this allows for fine silts, sands, and mud to settle out. Lagoons can become host to an anaerobic environment. This will allow high amounts of organic rich mud to form. Vegetation is also common.
Costal Hazards
Natural geomorphic processes are considered hazards when human populations are affected by them. For example, storms, hurricanes, and tsunamis are natural driving forces of coastal processes and landforms, but also cause loss of life and property in coastal communities. Storms provide much of the sediment to shallow-marine and estuarine ecosystems. Many wetland environments and barrier islands depend on storm activities for sediment build-up and survival when faced with rising sea levels. When combined with increasing sea levels, these events may have tremendous impacts on coastal environments and beaches.
Rip Currents
Rip currents are strong and swift, moving 3 to 6 feet (1 to 2 m) per second, which is faster than an average person can swim! These currents are created because of “set-up” near the shoreline. Set-up is a slight increase in water levels compared to those found seaward of the surf zone and creates unstable conditions that eventually are relieved through the formation of rip currents. These dangerous currents generally form at a low point or saddle in a sandbar. Because rip currents tend to be narrow, swimmers caught in a rip current should swim parallel to the shore to escape being taken out to sea. Swimmers should be especially cautious during storm events, which may increase the frequency and strength of rip currents.
Storm Surges
Storm surges—extraordinarily high water levels—are generated by the combined effects of low atmospheric pressure and very high wind speeds. The strong onshore winds that accompany tropical storms, hurricanes, and frontal storms of the midlatitudes drag and stack water against coasts, creating a storm surge. In the center of tropical-storm systems, atmospheric pressure may drop as much as 100 millibars below normal, and this can “suck up” sea level below the center of the cyclone by as much as 3.3 feet (1 m) (Summerfield 1991). Winds blowing toward coastal embayments produce the largest storm surges, which are accentuated if they coincide with high tides. By the time the waves generated by the storm surge reach the coast, they may have built up to a height of nearly 10 feet (several meters) above normal high tides.
When low-pressure storm systems approach land, strong winds can affect a coast in a variety of ways. High velocity onshore winds, particularly the kind associated with hurricanes, drive water ashore and elevate the water line well above the predicted tidal variation. The effect of storm surges can be catastrophic because the elevated water surface results in widespread coastal flooding and allows waves to break much further inland than they would normally. In addition, torrential rainfall associated with the storm causes widespread river flooding. The combination of these effects can result in extensive property damage and loss of life (Pinet 1992).
Although comparatively uncommon on a global basis, storm surges occur repeatedly along coasts experiencing tropical cyclones and in midlatitude areas subject to intense storms where the coastal configuration is particularly favorable. Storm surges are most destructive along very low-lying coasts where their effects can extend many miles (kilometers) inland, but their geomorphic significance arises in large part from the way in which they lead to wave attack at much higher levels along a shoreline than is reached by normal waves.
For more information about hurricanes, check out these Web sites:
- NASA satellite photos
- NASA “recipe for a hurricane”
- NASA information on Hurricane Isabel
- Story and photos of Hurricane Isabel by Hurricane Hunters
- Listing of more Web sites on hurricanes
- Hurricane Hunters home page
Tsunamis
Tsunamis are very large seismic ocean waves that are radially generated from volcanic eruptions, earthquakes, or subaqueous slumping. In the open ocean, these waves may travel at speeds in excess of 493 miles per hour (793 kph)! Strangely, sailors on deep ocean vessels may not notice the passing of these waves on account of the waves’ flat, low propagation. In contrast, when tsunamis reach shallow water, they slow down considerably and may reach great heights [up to 33 ft (10 m)]. Tsunamis have caused great destruction and loss of life because of abrupt changes in water levels above the normal high water mark. Numerous areas in the United States have experienced tsunamis including Hawaii, the Pacific Northwest, and Alaska. A new system, the International Tsunami Warning System, is used now to alert the public to impending tsunamis.
Use these Web links for more information about tsunamis: where they occur, why they occur, and what happens in a tsunami.
- Local tsunamis of the Pacific Northwest—USGS
- Tsunami and Earthquake Links—USGS
- Backgrounder: Tsunami—FEMA
- Tsunami: the Great Waves—NOAA
Human Modifications of Coastal Processes
People love living near the beach. More than 50% of the U.S. population lives within 50 miles (80 km) of a shoreline. Once developed, communities make an effort to protect their beach homes and coastal businesses. Throughout history, humans have attempted to slow or alter the dynamic coastal zone. The anthropogenic (human-influenced) changes to coastal environments may take many forms: creation or stabilization of inlets, beach nourishment and sediment bypassing, creation of dunes for property protection, dredging of waterways for shipping and commerce, and introduction of hard structures such as jetties, groins, and seawalls. These modifications change coastal features and have far-reaching effects on coastal processes and ecosystems. An understanding of how human changes alter shoreline environments and park resources is vital for the protection and preservation of coastal areas.
Variations in sea levels are natural responses to climate change, geodetic variations, movements of the sea floor, and other Earth processes. Human actions, including drainage of wetlands, withdrawal of groundwater (which eventually flows to the sea), and deforestation (which reduces terrestrial water-storage capacity), may also contribute to global rise in sea level. Additionally, human-induced climate change, primarily through the burning of fossil fuels, is also of importance. Local changes may be caused by large engineering works nearby, such as river channeling or dam construction that influence sediment delivery and deposition in deltaic areas.
The National Park Service allows natural coastal processes to continue without interference. However, when natural processes, including coastal erosion and storm events, interfere with the preservation of cultural resources and park infrastructure, modifications to coastal dynamics may be necessary. How coastal modifications will affect natural park resources (biological and physical) must be investigated thoroughly in order that wise decisions are made. Park managers in coastal parks strive to achieve a balance between preservation of historic landmarks (e.g., forts and lighthouses) and the protection of natural ecosystems. In addition, a history of long-term human alteration, combined with a lack of historical documentation, makes defining a natural coastal system difficult. An understanding of how anthropogenic modifications will alter shoreline environments and park resources is vital for effective coastal management.
Soft Structures
Dredging
Dredging, the removal of sediment, including sand, silt, rock, and other subaqueous materials from our coastal waterways is a hotly debated topic in coastal management. The effects of dredging waterways and ports to benefit shipping, transport, and recreation are not fully understood. Opponents claim that coastal dredging may have detrimental environmental impacts and may interfere with sediment transport and flow dynamics in coastal and marine systems.
Dredged sediments may include harmful contaminants and pollutants. After dredging, these sediments are often redeposited offshore or used for the creation of dredge spoil islands adjacent to the scoured waterways. In addition, dredged sediment may be incorporated into beach nourishment projects; however, the sediment grain size of dredged materials may not be compatible with native beach sediment. Grain-size alterations and contamination may exceed flora and fauna tolerances, negatively impacting native ecosystem functions.
Proponents of dredging cite that this method of removal is necessary for commerce, recreation, and national defense. Interagency partnerships, such as the Nature Conservancy and the U.S. Army Corps of Engineers, have been established to promote a better understanding of how dredging could impact coastal environments.
For more information about dredging, check out the Web sites:
- Information on dredging research, benefits, and resources; Dredging Operations Technical Support (DOTS) provides direct environmental and engineering support to the U.S. Army Corps of Engineers’ Operations and Maintenance (O&M) dredging missions
- Overview of dredging by the Monterey Bay National Marine Sanctuary (NOAA)
Beach Nourishment
Beach nourishment is the process of placing additional sediment on a beach. This material is obtained from another source that either lies inland or is dredged offshore. Nourishment entails the removal of sediment from “borrow sites,” and the subsequent transport of the sediment to beach areas. Borrow sites may alter sediment transport, hydrodynamic patterns, marine ecosystems, and sediment transport, such as creating erosional “hot spots” on adjacent shorelines.
Subaqueous nourishment is an alternative form of replenishment. The creation of offshore berms (mounds) may be utilized for the subsequent landward migration of sediments, often leading to sediment accretion on adjacent beaches. Although still a fairly new replenishment method, and not documented as fully effective, subaqueous nourishment may be substituted on account of cost limitations or biotic complications (e.g., migration and preserving endangered species) resulting from direct beach nourishment.
Often, beach nourishment is needed to counteract the effects of the hard-structure stabilization of coastlines. These structures (e.g., jetties, groins, and seawalls) typically increase downdrift erosion rates, promoting a need for continued coastal modifications through nourishment. The need for beach nourishment after human alteration is quite evident at Assateague Island National Seashore (Maryland). In the mid-1930s, the U.S. Army Corps of Engineers established a jetty system to stabilize the Ocean City inlet. While sediment transported by north-to-south longshore currents increased sediment accretion updrift of the jetties at Ocean City, excessive erosion and barrier island migration have occurred on the island to the south of the jetties. That is, Assateague Island has migrated westward more than 1,148 feet (350 m) since 1933! The deterrence of longshore sediment transport to Assateague Island National Park has had numerous detrimental impacts on biological, geologic, and cultural resources. Both short-term and long-term beach nourishment plans are in place to mitigate the destructive effects of jetty placement.
For more information about Assateague Island National Seashore, check out these Web sites:
- Ocean City–Assateague Island, Maryland, studies by Gregory Bass, U.S. Army Corps of Engineers
- Report by the U.S. Geological Survey on coastal conflicts that highlights Ocean City jetty construction and Assateague Island migration
- Article in Maryland Newsline that describes nourishment efforts by the U.S. Army Corps of Engineers on Assateague Island
For more information about methods, environmental impacts, and costs of beach nourishment, check out these Web sites:
- Overview of NOAA dredging activities, including legal and political constraints, sand resources for beach nourishment, and potential impacts of nourishment projects
- NOAA Web site containing a national overview of state, territory, and commonwealth beach nourishment programs
- Environmental review of beach nourishing projects by the U.S. Army Corps of Engineers
Beach Scraping
Beach scraping (i.e., grading and bulldozing) is the process of reshaping beach and dune landforms with heavy machinery. Usually a layer of sand from the lower beach is moved to the upper beach. Beach scraping creates dunes, which are used to give property owners some security from beach erosion, severe storms, and winter washover events. During the summers, the created sandbanks may be bulldozed flat, providing water views to property owners. However, the effects of beach scraping on coastal environments are little known, and this procedure may be harmful to coastal biota and habitats. Proponents claim that beach scraping is a time and cost-effective method to ensure shoreline protection, while opponents state that this method may be the most ecologically destructive form of coastal manipulation to date.
Please see this Web site for more information on beach scraping:
Hard Structures
Hard structures are often placed in marine environments to counteract erosion in sediment-deficient areas, or to deter accretion in sediment-rich areas such as inlets. Unfortunately, these anthropogenic modifications may accelerate erosion in adjacent downdrift areas, increasing the need for additional hard structures. The creation of new hard structures is currently banned in many states, or strongly discouraged as coastal management practice.
Groins are shore-perpendicular structures, used to maintain updrift beaches or to restrict longshore sediment transport. Permeable groins are becoming popular, and may negate some of the negative effects of impermeable groins. Another type of shore-perpendicular hard structure are jetties, which are normally placed adjacent to tidal inlets to control inlet migration and to minimize sediment deposition within the inlet.
Shore parallel structures include seawalls, bulkheads, and revetments. These structures are designed to protect coastal property. Development permits are relatively easy to obtain in many states because seawalls may be built above the high-water mark on private property, and they are relatively inexpensive, compared to beach nourishment. Ironically, seawalls usually accelerate erosion on beaches they are intended to protect. Wave energy is reflected off seawalls, increasing erosion in front of them. The placement of a seawall will decrease the sediment supply near seawalls, increasing erosion on adjacent beaches. In many areas, beaches have completely eroded and disappeared on account of seawalls.
Other anthropogenic structures that are used to stop or alter natural coastal changes include breakwaters, headlands, sills, and reefs. These structures are composed of either natural or artificial materials, and are designed to alter the effects of waves and slow coastline erosion and change. Submerged reefs and sills dampen wave energy and may create new habitat, which is significant for local fisheries. However, the long-term effects of these structures, on both physical and biological processes, are not understood and require thorough examination.
For more information concerning these and other anthropogenic modifications, check out these Web sites:
- Wilmington Morning Star article concerning the construction of hard structures on North Carolina shores; contains links to other articles discussing coastal management issues, Dr. Orrin Pilkey, and barrier-island geomorphology
- Associated Press article “Development-protecting seawalls debated”
Check Your Understanding
The Sun and the Moon control _________.
- currents.
- waves.
- tides.
Show Answer
tides.
Candela Citations
- Introduction to Oceans and Shorelines. Authored by: Kimberly Schulte and Lumen Learning. Provided by: Lumen Learning. License: CC BY: Attribution
- Revision and adaptation of Wikipedia content. Authored by: Kimberly Schulte and Lumen Learning. Provided by: Lumen Learning. License: CC BY-SA: Attribution-ShareAlike
- Waves in pacifica. Authored by: Brocken Inaglory. Located at: https://commons.wikimedia.org/wiki/File:Waves_in_pacifica_1.jpg. License: CC BY-SA: Attribution-ShareAlike
- Bay of Fundy High Tide. Authored by: Samuel Wantman. Provided by: Wikimedia Commons. Located at: https://commons.wikimedia.org/wiki/File:Bay_of_Fundy_High_Tide.jpg. License: CC BY-SA: Attribution-ShareAlike
- Bay of Fundy Low Tide. Authored by: Samuel Wantman. Provided by: Wikimedia Commons. Located at: https://commons.wikimedia.org/wiki/File:Bay_of_Fundy_Low_Tide.jpg. License: CC BY-SA: Attribution-ShareAlike
- Beach. Provided by: Wikipedia. Located at: https://en.wikipedia.org/wiki/Beach. License: CC BY-SA: Attribution-ShareAlike
- Cliffed coast. Provided by: Wikipedia. Located at: https://en.wikipedia.org/wiki/Cliffed_coast. License: CC BY-SA: Attribution-ShareAlike
- River delta. Provided by: Wikipedia. Located at: https://en.wikipedia.org/wiki/River_delta. License: CC BY-SA: Attribution-ShareAlike
- Reef. Provided by: Wikipedia. Located at: https://en.wikipedia.org/wiki/Reef. License: CC BY-SA: Attribution-ShareAlike
- Barrier island. Provided by: Wikipedia. Located at: https://en.wikipedia.org/wiki/Barrier_island. License: CC BY-SA: Attribution-ShareAlike
- Coastal Processes. Provided by: National Park Service. Located at: http://www.nature.nps.gov/views/KCs/CoastalG/HTML/ET_Processes.htm. Project: Views of the National Park. License: Public Domain: No Known Copyright
- Ocean surface currents. Provided by: NOAA. Located at: http://www.adp.noaa.gov/currents_map.html. License: Public Domain: No Known Copyright
- Whittow, John (1984). Dictionary of Physical Geography. London: Penguin, 1984, p. 97. See also Herbert Louis and Klaus Fischer: Allgemeine Geomorphologie, de Gruyter, 4th ed., Berlin 1979, pp. 532–537 ↵
- Slingerland, R. and N. D. Smith (1998), "Necessary conditions for a meandering-river avulsion," Geology (Boulder), 26, 435–438. ↵
- Jürgen Schieber, Possible indicators of microbial mat deposits in shales and sandstones: examples from the Mid-Proterozoic Belt Supergroup, Montana, U.S.A., Sedimentary Geology, 120 (1998) pp. 105–124 ↵
- Garrison, J.R., Jr., Williams, J., Potter Miller, S., Weber, E.T., II, McMechan, G., and Zeng, X., 2010, "Ground-penetrating radar study of North Padre Island; Implications for barrier island interval architecture, model for growth of progradational microtidal barrier islands, and Gulf of Mexico sea-level cyclicity:" Journal of Sedimentary Research, v. 80, p. 303–319. ↵
- Davis Jr., Richard A.; Fitzgerald, Duncan M. (2004), Beaches and Coasts, United Kingdom: Blackwell Publishing, p. 144. ↵
- Smith, Q.H.T., Heap, A.D., and Nichol, S.L., 2010, "Origin and formation of an estuarine barrier island, Tapora Island, New Zealand:" Journal of Coastal Research, v. 26, p. 292–300. ↵