Learning Objectives
By the end of this section, you will be able to:
- Describe how we can detect interstellar dust
- Understand the role and importance of infrared observations in studying dust
- Explain the terms extinction and interstellar reddening
Figure 1 shows a striking example of what is actually a common sight through large telescopes: a dark region on the sky that appears to be nearly empty of stars. For a long time, astronomers debated whether these dark regions were empty “tunnels” through which we looked beyond the stars of the Milky Way Galaxy into intergalactic space, or clouds of some dark material that blocked the light of the stars beyond. The astronomer William Herschel (discoverer of the planet Uranus) thought it was the former, once remarking after seeing one, “Here truly is a hole in heaven!” However, American astronomer E. E. Barnard is generally credited with showing from his extensive series of nebula photographs that the latter interpretation is the correct one (see the feature box on Edward Emerson Barnard).
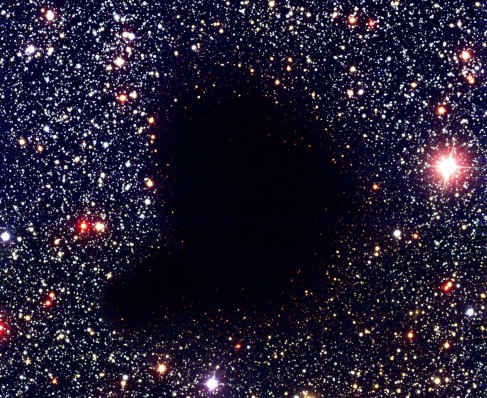
Figure 1. Barnard 68: This object, first catalogued by E. E. Barnard, is a dark interstellar cloud. Its striking appearance is due to the fact that, since it is relatively close to Earth, there are no bright stars between us and it, and its dust obscures the light from the stars behind it. (It looks a little bit like a sideways heart; one astronomers sent a photo of this object to his sweetheart as a valentine.) (credit: modification of work by ESO)
Dusty clouds in space betray their presence in several ways: by blocking the light from distant stars, by emitting energy in the infrared part of the spectrum, by reflecting the light from nearby stars, and by making distant stars look redder than they really are.
Edward Emerson Barnard
Born in 1857 in Nashville, Tennessee, two months after his father died, Edward Barnard (Figure 2) grew up in such poor circumstances that he had to drop out of school at age nine to help support his ailing mother. He soon became an assistant to a local photographer, where he learned to love both photography and astronomy, destined to become the dual passions of his life. He worked as a photographer’s aide for 17 years, studying astronomy on his own. In 1883, he obtained a job as an assistant at the Vanderbilt University Observatory, which enabled him at last to take some astronomy courses.
Married in 1881, Barnard built a house for his family that he could ill afford. But as it happened, a patent medicine manufacturer offered a $200 prize (a lot of money in those days) for the discovery of any new comet. With the determination that became characteristic of him, Barnard spent every clear night searching for comets. He discovered seven of them between 1881 and 1887, earning enough money to make the payments on his home; this “Comet House” later became a local attraction. (By the end of his life, Barnard had found 17 comets through diligent observation.)
In 1887, Barnard got a position at the newly founded Lick Observatory, where he soon locked horns with the director, Edward Holden, a blustering administrator who made Barnard’s life miserable. (To be fair, Barnard soon tried to do the same for him.) Despite being denied the telescope time that he needed for his photographic work, in 1892, Barnard managed to discover the first new moon found around Jupiter since Galileo’s day, a stunning observational feat that earned him world renown. Now in a position to demand more telescope time, he perfected his photographic techniques and soon began to publish the best images of the Milky Way taken up to that time. It was during the course of this work that he began to examine the dark regions among the crowded star lanes of the Galaxy and to realize that they must be vast clouds of obscuring material (rather than “holes” in the distribution of stars).
Astronomer-historian Donald Osterbrock has called Barnard an “observaholic:” his daily mood seemed to depend entirely on how clear the sky promised to be for his night of observing. He was a driven, neurotic man, concerned about his lack of formal training, fearful of being scorned, and afraid that he might somehow slip back into the poverty of his younger days. He had difficulty taking vacations and lived for his work: only serious illness could deter him from making astronomical observations.
In 1895, Barnard, having had enough of the political battles at Lick, accepted a job at the Yerkes Observatory near Chicago, where he remained until his death in 1923. He continued his photographic work, publishing compilations of his images that became classic photographic atlases, and investigating the varieties of nebulae revealed in his photographs. He also made measurements of the sizes and features of planets, participated in observations of solar eclipses, and carefully cataloged dark nebulae (see Figure 1). In 1916, he discovered the star with the largest proper motion, the second-closest star system to our own (see Analyzing Starlight). It is now called Barnard’s Star in his honor.
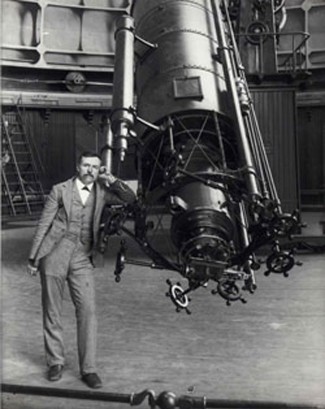
Figure 2. Edward Emerson Barnard (1857–1923): Barnard’s observations provided information that furthered many astronomical explorations. (credit: The Lick Observatory)
Detecting Dust
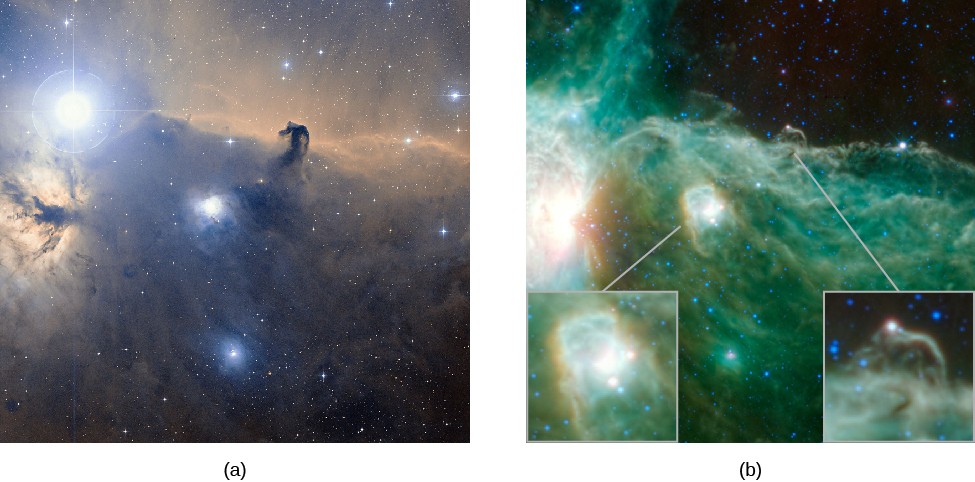
Figure 3. Visible and Infrared Images of the Horsehead Nebula in Orion. At left, (a) is a visible light image of the
The dark cloud seen in Figure 1 blocks the light of the many stars that lie behind it; note how the regions in other parts of the photograph are crowded with stars. Barnard 68 is an example of a relatively dense cloud or dark nebula containing tiny, solid dust grains. Such opaque clouds are conspicuous on any photograph of the Milky Way, the galaxy in which the Sun is located (see the figures in The Milky Way Galaxy). The “dark rift,” which runs lengthwise down a long part of the Milky Way in our sky and appears to split it in two, is produced by a collection of such obscuring clouds.
While dust clouds are too cold to radiate a measurable amount of energy in the visible part of the spectrum, they glow brightly in the infrared (Figure 2). The reason is that small dust grains absorb visible light and ultraviolet radiation very efficiently. The grains are heated by the absorbed radiation, typically to temperatures from 10 to about 500 K, and re-radiate this heat at infrared wavelengths.
Thanks to their small sizes and low temperatures, interstellar grains radiate most of their energy at infrared to microwave frequencies, with wavelengths of tens to hundreds of microns. Earth’s atmosphere is opaque to radiation at these wavelengths, so emission by interstellar dust is best measured from space. Observations from above Earth’s atmosphere show that dust clouds are present throughout the plane of the Milky Way (Figure 4).
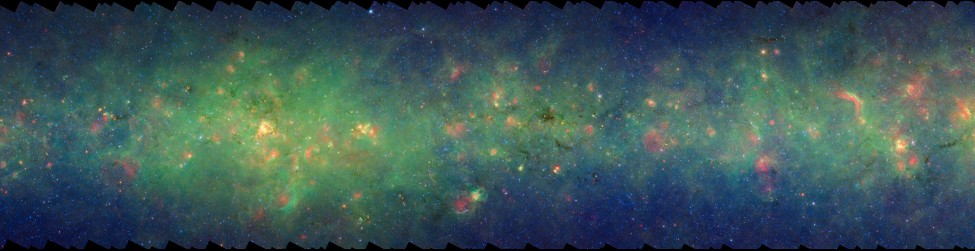
Figure 4. Infrared Emission from the Plane of the Milky Way: This infrared image taken by the Spitzer Space Telescope shows a field in the plane of the Milky Way Galaxy. (Our Galaxy is in the shape of a frisbee; the plane of the Milky Way is the flat disk of that frisbee. Since the Sun, Earth, and solar system are located in the plane of the Milky Way and at a large distance from its center, we view the Galaxy edge on, much as we might look at a glass plate from its edge.) This emission is produced by tiny dust grains, which emit at 3.6 microns (blue in this image), 8.0 microns (green), and 24 microns (red). The densest regions of dust are so cold and opaque that they appear as dark clouds even at these infrared wavelengths. The red bubbles visible throughout indicate regions where the dust has been warmed up by young stars. This heating increases the emission at 24 microns, leading to the redder color in this image. (credit: modification of work by NASA/JPL-Caltech/University of Wisconsin)
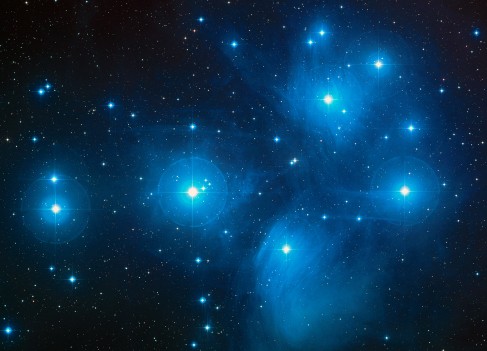
Figure 5. Pleiades Star Cluster: The bluish light surrounding the stars in this image is an example of a reflection nebula. Like fog around a street lamp, a reflection nebula shines only because the dust within it scatters light from a nearby bright source. The Pleiades cluster is currently passing through an interstellar cloud that contains dust grains, which scatter the light from the hot blue stars in the cluster. The Pleiades cluster is about 400 light-years from the Sun. (credit: NASA, ESA and AURA/Caltech)
Some dense clouds of dust are close to luminous stars and scatter enough starlight to become visible. Such a cloud of dust, illuminated by starlight, is called a reflection nebula, since the light we see is starlight reflected off the grains of dust. One of the best-known examples is the nebulosity around each of the brightest stars in the Pleiades cluster. The dust grains are small, and such small particles turn out to scatter light with blue wavelengths more efficiently than light at red wavelengths. A reflection nebula, therefore, usually appears bluer than its illuminating star (Figure 5).
Gas and dust are generally intermixed in space, although the proportions are not exactly the same everywhere. The presence of dust is apparent in many photographs of emission nebulae in the constellation of Sagittarius, where we see an H II region surrounded by a blue reflection nebula. Which type of nebula appears brighter depends on the kinds of stars that cause the gas and dust to glow. Stars cooler than about 25,000 K have so little ultraviolet radiation of wavelengths shorter than 91.2 nanometers—which is the wavelength required to ionize hydrogen—that the reflection nebulae around such stars outshine the emission nebulae. Stars hotter than 25,000 K emit enough ultraviolet energy that the emission nebulae produced around them generally outshine the reflection nebulae.
Interstellar Reddening
The tiny interstellar dust grains absorb some of the starlight they intercept. But at least half of the starlight that interacts with a grain is merely scattered, that is, it is redirected rather than absorbed. Since neither the absorbed nor the scattered starlight reaches us directly, both absorption and scattering make stars look dimmer. The effects of both processes are called interstellar extinction (Figure 6).
Astronomers first came to understand interstellar extinction around the early 1930s, as the explanation of a puzzling observation. In the early part of the twentieth century, astronomers discovered that some stars look red even though their spectral lines indicate that they must be extremely hot (and thus should look blue). The solution to this seeming contradiction turned out to be that the light from these hot stars is not only dimmed but also reddened by interstellar dust, a phenomenon known as interstellar reddening.
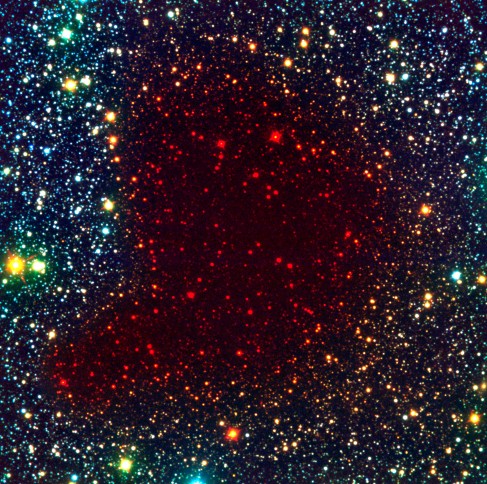
Figure 6. Barnard 68 in Infrared: In this image, we see Barnard 68, the same object shown in [link]. The difference is that, in the previous image, the blue, green, and red channels showed light in the visible (or very nearly visible) part of the spectrum. In this image, the red color shows radiation emitted in the infrared at a wavelength of 2.2 microns. Interstellar extinction is much smaller at infrared than at visible wavelengths, so the stars behind the cloud become visible in the infrared channel. (credit: ESO)
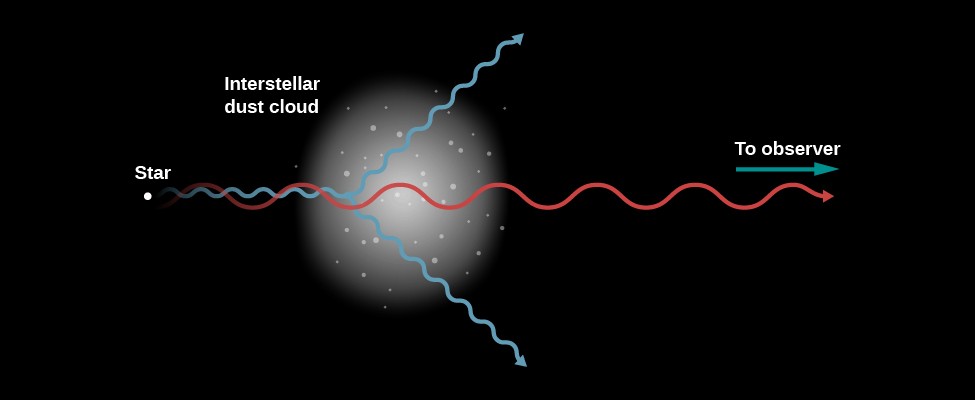
Figure 6. Scattering of Light by Dust: Interstellar dust scatters blue light more efficiently than red light, thereby making distant stars appear redder and giving clouds of dust near stars a bluish hue. Here, a red ray of light from a star comes straight through to the observer, whereas a blue ray is shown scattering. A similar scattering process makes Earth’s sky look blue.
Dust does not interact with all the colors of light the same way. Much of the violet, blue, and green light from these stars has been scattered or absorbed by dust, so it does not reach Earth. Some of their orange and red light, with longer wavelengths, on the other hand, more easily penetrates the intervening dust and completes its long journey through space to enter Earth-based telescopes (Figure 7). Thus, the star looks redder from Earth than it would if you could see it from nearby. (Strictly speaking, reddening is not the most accurate term for this process, since no red color is added; instead, blues and related colors are subtracted, so it should more properly be called “deblueing.”) In the most extreme cases, stars can be so reddened that they are entirely undetectable at visible wavelengths and can be seen only at infrared or longer wavelengths (Figure 6).
We have all seen an example of reddening on Earth. The Sun appears much redder at sunset than it does at noon. The lower the Sun is in the sky, the longer the path its light must travel through the atmosphere. Over this greater distance, there is a greater chance that sunlight will be scattered. Since red light is less likely to be scattered than blue light, the Sun appears more and more red as it approaches the horizon.
By the way, scattering of sunlight is also what causes our sky to look blue, even though the gases that make up Earth’s atmosphere are transparent. As sunlight comes in, it scatters from the molecules of air. The small size of the molecules means that the blue colors scatter much more efficiently than the greens, yellows, and reds. Thus, the blue in sunlight is scattered out of the beam and all over the sky. The light from the Sun that comes to your eye, on the other hand, is missing some of its blue, so the Sun looks a bit yellower, even when it is high in the sky, than it would from space.
The fact that starlight is reddened by interstellar dust means that long-wavelength radiation is transmitted through the Galaxy more efficiently than short-wavelength radiation. Consequently, if we wish to see farther in a direction with considerable interstellar material, we should look at long wavelengths. This simple fact provides one of the motivations for the development of infrared astronomy. In the infrared region at 2 microns (2000 nanometers), for example, the obscuration is only one-sixth as great as in the visible region (500 nanometers), and we can therefore study stars that are more than twice as distant before their light is blocked by interstellar dust. This ability to see farther by observing in the infrared portion of the spectrum represents a major gain for astronomers trying to understand the structure of our Galaxy or probing its puzzling, but distant, center (see The Milky Way Galaxy).
Interstellar Grains
Before we get to the details about interstellar dust, we should perhaps get one concern out of the way. Why couldn’t it be the interstellar gas that reddens distant stars and not the dust? We already know from everyday experience that atomic or molecular gas is almost transparent. Consider Earth’s atmosphere. Despite its very high density compared with that of interstellar gas, it is so transparent as to be practically invisible. (Gas does have a few specific spectral lines, but they absorb only a tiny fraction of the light as it passes through.)The quantity of gas required to produce the observed absorption of light in interstellar space would have to be enormous. The gravitational attraction of so great a mass of gas would affect the motions of stars in ways that could easily be detected. Such motions are not observed, and thus, the interstellar absorption cannot be the result of gases.
Although gas does not absorb much light, we know from everyday experience that tiny solid or liquid particles can be very efficient absorbers. Water vapor in the air is quite invisible. When some of that vapor condenses into tiny water droplets, however, the resulting cloud is opaque. Dust storms, smoke, and smog offer familiar examples of the efficiency with which solid particles absorb light. On the basis of arguments like these, astronomers have concluded that widely scattered solid particles in interstellar space must be responsible for the observed dimming of starlight. What are these particles made of? And how did they form?
Observations like the pictures in this chapter show that a great deal of this dust exists; hence, it must be primarily composed of elements that are abundant in the universe (and in interstellar matter). After hydrogen and helium, the most abundant elements are oxygen, carbon, and nitrogen. These three elements, along with magnesium, silicon, iron—and perhaps hydrogen itself—turn out to be the most important components of interstellar dust.
Many of the dust particles can be characterized as sootlike (rich in carbon) or sandlike (containing silicon and oxygen). Grains of interstellar dust are found in meteorites and can be identified because the abundances of certain isotopes are different from what we see in other solar system material. Several different interstellar dust substances have been identified in this way in the laboratory, including graphite and diamonds. (Don’t get excited; these diamonds are only a billionth of a meter in size and would hardly make an impressive engagement ring!)
The most widely accepted model pictures the grains with rocky cores that are either like soot (rich in carbon) or like sand (rich in silicates). In the dark clouds where molecules can form, these cores are covered by icy mantles (Figure 7). The most common ices in the grains are water (H2O), methane (CH4), and ammonia (NH3)—all built out of atoms that are especially abundant in the realm of the stars. The ice mantles, in turn, are sites for some of the chemical reactions that produce complex organic molecules.
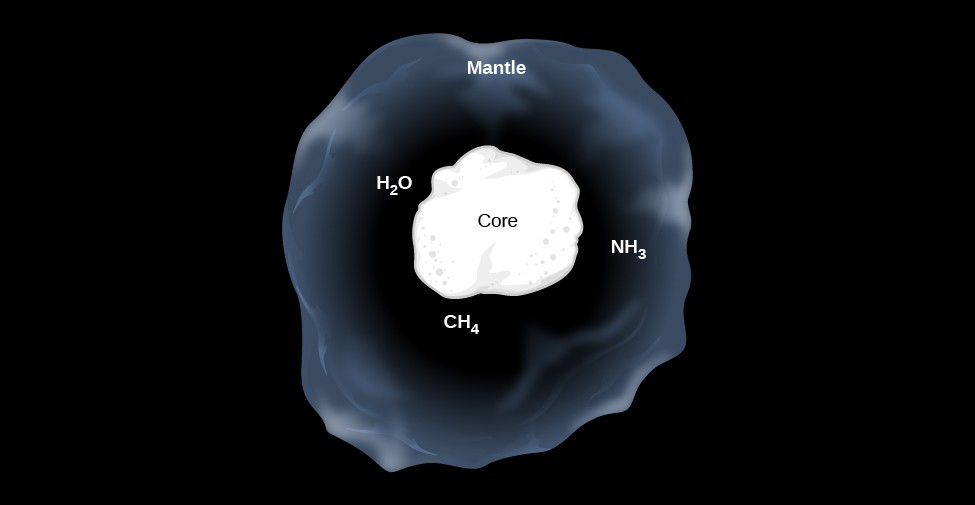
Figure 7. Model of an Interstellar Dust Grain: A typical interstellar grain is thought to consist of a core of rocky material (silicates) or graphite, surrounded by a mantle of ices. Typical grain sizes are 10–8 to 10–7 meters. (This is from 1/100 to 1/10 of a micron; by contrast, human hair is about 10–200 microns wide.)
Typical individual grains must be just slightly smaller than the wavelength of visible light. If the grains were a lot smaller, they would not block the light efficiently.
On the other hand, if the dust grains were much larger than the wavelength of light, then starlight would not be reddened. Things that are much larger than the wavelength of light would block both blue and red light with equal efficiency. In this way we can deduce that a characteristic interstellar dust grain contains 106 to 109 atoms and has a diameter of 10–8 to 10–7 meters (10 to 100 nanometers). This is actually more like the specks of solid matter in cigarette smoke than the larger grains of dust you might find under your desk when you are too busy studying astronomy to clean properly.
Key Concepts and Summary
Interstellar dust can be detected: (1) when it blocks the light of stars behind it, (2) when it scatters the light from nearby stars, and (3) because it makes distant stars look both redder and fainter. These effects are called reddening and interstellar extinction, respectively. Dust can also be detected in the infrared because it emits heat radiation. Dust is found throughout the plane of the Milky Way. The dust particles are about the same size as the wavelength of light and consist of rocky cores that are either sootlike (carbon-rich) or sandlike (silicates) with mantles made of ices such as water, ammonia, and methane.
Glossary
interstellar extinction: the attenuation or absorption of light by dust in the interstellar medium
reddening (interstellar): the reddening of starlight passing through interstellar dust because dust scatters blue light more effectively than red
Candela Citations
- Astronomy. Provided by: OpenStax CNX. Located at: http://cnx.org/contents/2e737be8-ea65-48c3-aa0a-9f35b4c6a966@10.1. License: CC BY: Attribution. License Terms: Download for free at http://cnx.org/contents/2e737be8-ea65-48c3-aa0a-9f35b4c6a966@10.1.