Learning Objectives
By the end of this section, you will be able to:
- Describe the characteristics common to all quasars
- Justify the claim that supermassive black holes are the source of the energy emitted by quasars (and AGNs)
- Explain how a quasar’s energy is produced
In order to find a common model for quasars (and their cousins, the AGNs), let’s first list the common characteristics we have been describing and add some new ones:
- Quasars are hugely powerful, emitting more power in radiated light than all the stars in our Galaxy combined.
- Quasars are tiny, about the size of our solar system (to astronomers, that is really small!).
- Some quasars are observed to be shooting out pairs of straight jets at close to the speed of light, in a tight beam, to distances far beyond the galaxies they live in. These jets are themselves powerful sources of radio and gamma-ray radiation.
- Because quasars put out so much power from such a small region, they can’t be powered by nuclear fusion the way stars are; they must use some process that is far more efficient.
- As we shall see later in this chapter, quasars were much more common when the universe was young than they are today. That means they must have been able to form in the first billion years or so after the universe began to expand.
The readers of this text are in a much better position than the astronomers who discovered quasars in the 1960s to guess what powers the quasars. That’s because the key idea in solving the puzzle came from observations of the black holes. The discovery of the first stellar mass black hole in the binary system Cygnus X-1 was announced in 1971, several years after the discovery of quasars. Proof that there is a black hole at the center of our own Galaxy came even later. Back when astronomers first began trying to figure out what powered quasars, black holes were simply one of the more exotic predictions of the general theory of relativity that still waited to be connected to the real world.
It was only as proof of the existence of black holes accumulated over several decades that it became clearer that only supermassive black holes could account for all the observed properties of quasars and AGNs. As we saw in The Milky Way Galaxy, our own Galaxy has a black hole in its center, and the energy is emitted from a small central region. While our black hole doesn’t have the mass or energy of the quasar black holes, the mechanism that powers them is similar. The evidence now shows that most—and probably all—elliptical galaxies and all spirals with nuclear bulges have black holes at their centers. The amount of energy emitted by material near the black hole depends on two things: the mass of the black hole and the amount of matter that is falling into it.
If a black hole with a billion Suns’ worth of mass inside (109MSun) accretes (gathers) even a relatively modest amount of additional material—say, about 10 MSun per year—then (as we shall see) it can, in the process, produce as much energy as a thousand normal galaxies. This is enough to account for the total energy of a quasar. If the mass of the black hole is smaller than a billion solar masses or the accretion rate is low, then the amount of energy emitted can be much smaller, as it is in the case of the Milky Way.
Observational Evidence for Black Holes
In order to prove that a black hole is present at the center of a galaxy, we must demonstrate that so much mass is crammed into so small a volume that no normal objects—massive stars or clusters of stars—could possibly account for it (just as we did for the black hole in the Milky Way). We already know from observations (discussed in Black Holes and Curved Spacetime) that an accreting black hole is surrounded by a hot accretion disk with gas and dust that swirl around the black hole before it falls in.
If we assume that the energy emitted by quasars is also produced by a hot accretion disk, then, as we saw in the previous section, the size of the disk must be given by the time the quasar energy takes to vary. For quasars, the emission in visible light varies on typical time scales of 5 to 2000 days, limiting the size of the disk to that many light-days.
In the X-ray band, quasars vary even more rapidly, so the light travel time argument tells us that this more energetic radiation is generated in an even smaller region. Therefore, the mass around which the accretion disk is swirling must be confined to a space that is even smaller. If the quasar mechanism involves a great deal of mass, then the only astronomical object that can confine a lot of mass into a very small space is a black hole. In a few cases, it turns out that the X-rays are emitted from a region just a few times the size of the black hole event horizon.
The next challenge, then, is to “weigh” this central mass in a quasar. In the case of our own Galaxy, we used observations of the orbits of stars very close to the galactic center, along with Kepler’s third law, to estimate the mass of the central black hole (The Milky Way Galaxy). In the case of distant galaxies, we cannot measure the orbits of individual stars, but we can measure the orbital speed of the gas in the rotating accretion disk. The Hubble Space Telescope is especially well suited to this task because it is above the blurring of Earth’s atmosphere and can obtain spectra very close to the bright central regions of active galaxies. The Doppler effect is then used to measure radial velocities of the orbiting material and so derive the speed with which it moves around.
One of the first galaxies to be studied with the Hubble Space Telescope is our old favorite, the giant elliptical M87. Hubble Space Telescope images showed that there is a disk of hot (10,000 K) gas swirling around the center of M87 (Figure 1). It was surprising to find hot gas in an elliptical galaxy because this type of galaxy is usually devoid of gas and dust. But the discovery was extremely useful for pinning down the existence of the black hole. Astronomers measured the Doppler shift of spectral lines emitted by this gas, found its speed of rotation, and then used the speed to derive the amount of mass inside the disk—applying Kepler’s third law.
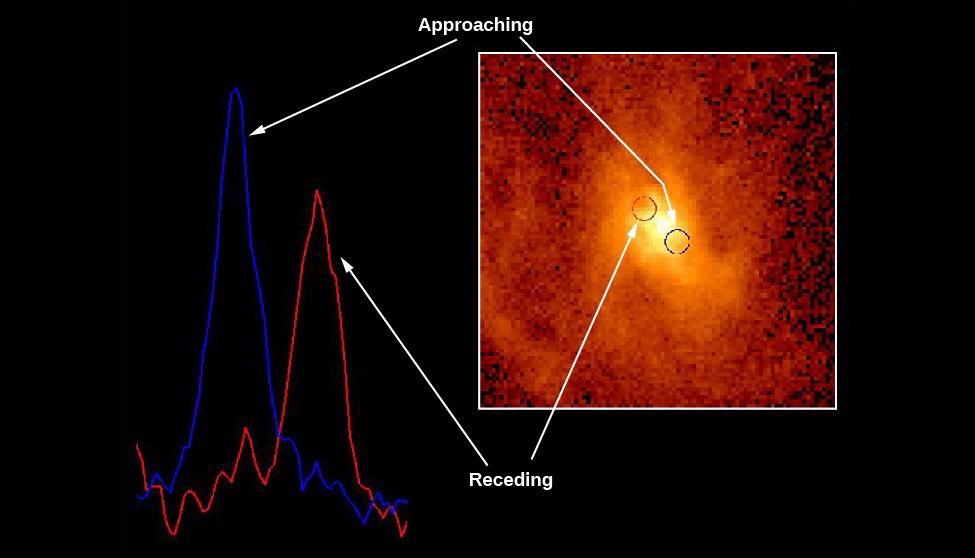
Figure 1. Evidence for a Black Hole at the Center of M87: The disk of whirling gas at right was discovered at the center of the giant elliptical galaxy M87 with the Hubble Space Telescope. Observations made on opposite sides of the disk show that one side is approaching us (the spectral lines are blueshifted by the Doppler effect) while the other is receding (lines redshifted), a clear indication that the disk is rotating. The rotation speed is about 550 kilometers per second or 1.2 million miles per hour. Such a high rotation speed is evidence that there is a very massive black hole at the center of M87. (credit: modification of work by Holland Ford, STScI/JHU; Richard Harms, Linda Dressel, Ajay K. Kochhar, Applied Research Corp.; Zlatan Tsvetanov, Arthur Davidsen, Gerard Kriss, Johns Hopkins; Ralph Bohlin, George Hartig, STScI; Bruce Margon, University of Washington in Seattle; NASA)
Modern estimates show that there is a mass of at least 3.5 billion MSun concentrated in a tiny region at the very center of M87. So much mass in such a small volume of space must be a black hole. Let’s stop for a moment and take in this figure: a single black hole that has swallowed enough material to make 3.5 billion stars like the Sun. Few astronomical measurements have ever led to so mind-boggling a result. What a strange environment the neighborhood of such a supermassive black hole must be.
Another example is shown in Figure 2. Here, we see a disk of dust and gas that surrounds a 300-million-MSun black hole in the center of an elliptical galaxy. (The bright spot in the center is produced by the combined light of stars that have been pulled close together by the gravitational force of the black hole.) The mass of the black hole was again derived from measurements of the rotational speed of the disk. The gas in the disk is moving around at 155 kilometers per second at a distance of only 186 light-years from its center. Given the pull of the mass at the center, we expect that the whole dust disk should be swallowed by the black hole in several billion years.
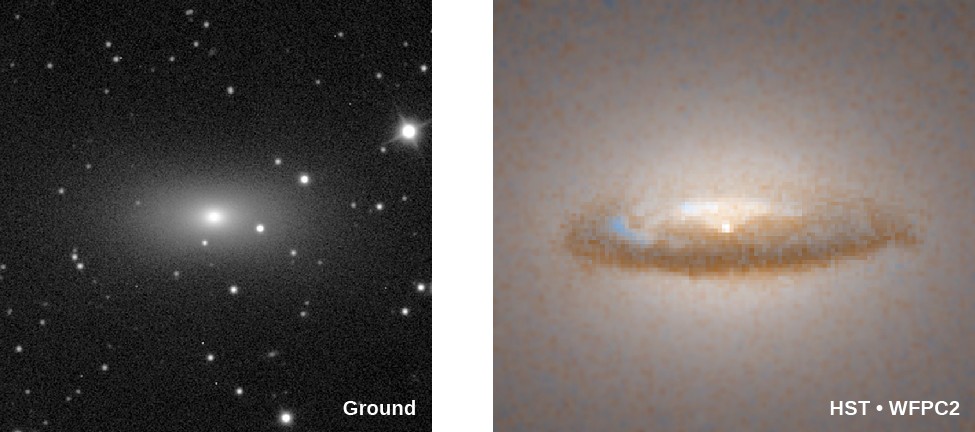
Figure 2. Another Galaxy with a Black-Hole Disk: The ground-based image shows an elliptical galaxy called NGC 7052 located in the constellation of Vulpecula, almost 200 million light-years from Earth. At the galaxy’s center (right) is a dust disk roughly 3700 light-years in diameter. The disk rotates like a giant merry-go-round: gas in the inner part (186 light-years from the center) whirls around at a speed of 155 kilometers per second (341,000 miles per hour). From these measurements and Kepler’s third law, it is possible to estimate that the disk is orbiting around a central black hole with a mass of 300 million Suns. (credit: modification of work by Roeland P. van der Marel (STScI), Frank C. van den Bosch (University of Washington), NASA)
But do we have to accept black holes as the only explanation of what lies at the center of these galaxies? What else could we put in such a small space other than a giant black hole? The alternative is stars. But to explain the masses in the centers of galaxies without a black hole we need to put at least a million stars in a region the size of the solar system. To fit, they would have be only 2 star diameters apart. Collisions between stars would happen all the time. And these collisions would lead to mergers of stars, and very soon the one giant star that they form would collapse into a black hole. So there is really no escape: only a black hole can fit so much mass into so small a space.
As we saw earlier, observations now show that all the galaxies with a spherical concentration of stars—either elliptical galaxies or spiral galaxies with nuclear bulges (see the chapter on Galaxies)—harbor one of these giant black holes at their centers. Among them is our neighbor spiral galaxy, the Andromeda galaxy, M31. The masses of these central black holes range from a just under a million up to at least 30 billion times the mass of the Sun. Several black holes may be even more massive, but the mass estimates have large uncertainties and need verification. We call these black holes “supermassive” to distinguish them from the much smaller black holes that form when some stars die (see The Death of Stars). So far, the most massive black holes from stars—those detected through gravitational waves detected by LIGO—have masses only a little over 30 solar masses.
Energy Production around a Black Hole
By now, you may be willing to entertain the idea that huge black holes lurk at the centers of active galaxies. But we still need to answer the question of how such a black hole can account for one of the most powerful sources of energy in the universe. As we saw in Black Holes and Curved Spacetime, a black hole itself can radiate no energy. Any energy we detect from it must come from material very close to the black hole, but not inside its event horizon.
In a galaxy, a central black hole (with its strong gravity) attracts matter—stars, dust, and gas—orbiting in the dense nuclear regions. This matter spirals in toward the spinning black hole and forms an accretion disk of material around it. As the material spirals ever closer to the black hole, it accelerates and becomes compressed, heating up to temperatures of millions of degrees. Such hot matter can radiate prodigious amounts of energy as it falls in toward the black hole.
To convince yourself that falling into a region with strong gravity can release a great deal of energy, imagine dropping a printed version of your astronomy textbook out the window of the ground floor of the library. It will land with a thud, and maybe give a surprised pigeon a nasty bump, but the energy released by its fall will not be very great. Now take the same book up to the fifteenth floor of a tall building and drop it from there. For anyone below, astronomy could suddenly become a deadly subject; when the book hits, it does so with a great deal of energy.
Dropping things from far away into the much stronger gravity of a black hole is much more effective in turning the energy released by infall into other forms of energy. Just as the falling book can heat up the air, shake the ground, or produce sound energy that can be heard some distance away, so the energy of material falling toward a black hole can be converted to significant amounts of electromagnetic radiation.
What a black hole has to work with is not textbooks but streams of infalling gas. If a dense blob of gas moves through a thin gas at high speed, it heats up as it slows by friction. As it slows down, kinetic (motion) energy is turned into heat energy. Just like a spaceship reentering the atmosphere (Figure 3) gas approaching a black hole heats up and glows where it meets other gas. But this gas, as it approaches the event horizon, reaches speeds of 10% the speed of light and more. It therefore gets far, far hotter than a spaceship, which reaches no more than about 1500 K. Indeed, gas near a supermassive black hole reaches a temperature of about 150,000 K, about 100 times hotter than a spaceship returning to Earth. It can even get so hot—millions of degrees—that it radiates X-rays.
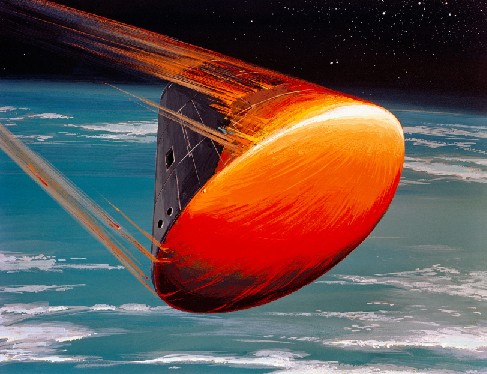
Figure 3. Friction in Earth’s Atmosphere: In this artist’s impression, the rapid motion of a spacecraft (the Apollo mission reentry capsule) through the atmosphere compresses and heats the air ahead of it, which heats the spacecraft in turn until it glows red hot. Pushing on the air slows down the spacecraft, turning the kinetic energy of the spacecraft into heat. Fast-moving gas falling into a quasar heats up in a similar way. (credit: modification of work by NASA)
The amount of energy that can be liberated this way is enormous. Einstein showed that mass and energy are interchangeable with his famous formula E = mc2 (see The Sun: A Nuclear Powerhouse). A hydrogen bomb releases just 1% of that energy, as does a star. Quasars are much more efficient than that. The energy released falling to the event horizon of a black hole can easily reach 10% or, in the extreme theoretical limit, 32%, of that energy. (Unlike the hydrogen atoms in a bomb or a star, the gas falling into the black hole is not actually losing mass from its atoms to free up the energy; the energy is produced just because the gas is falling closer and closer to the black hole.) This huge energy release explains how a tiny volume like the region around a black hole can release as much power as a whole galaxy. But to radiate all that energy, instead of just falling inside the event horizon with barely a peep, the hot gas must take the time to swirl around the star in the accretion disk and emit some of its energy.
Most black holes don’t show any signs of quasar emission. We call them “quiescent.” But, like sleeping dragons, they can be woken up by being roused with a fresh supply of gas. Our own Milky Way black hole is currently quiescent, but it may have been a quasar just a few million years ago (Figure 4). Two giant bubbles that extend 25,000 light-years above and below the galactic center are emitting gamma rays. Were these produced a few million years ago when a significant amount of matter fell into the black hole at the center of the galaxy? Astronomers are still working to understand what remarkable event might have formed these enormous bubbles.
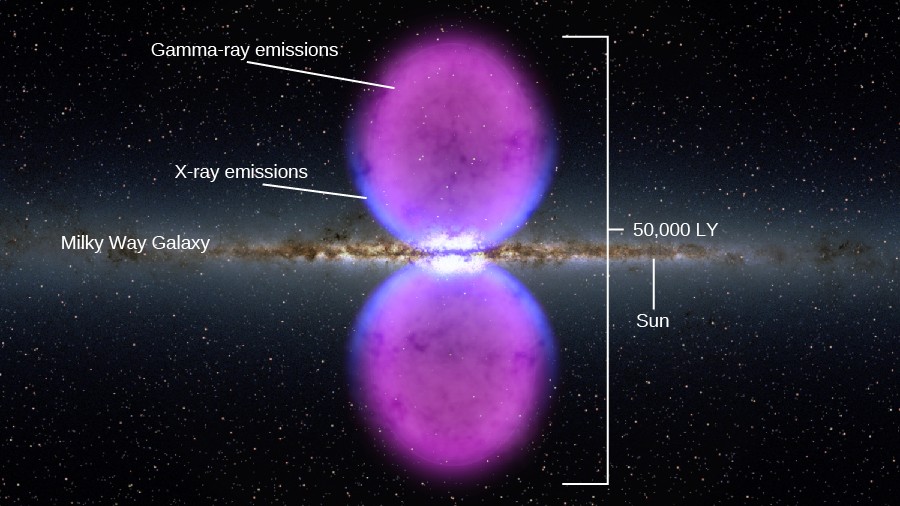
Figure 4. Fermi Bubbles in the Galaxy: Giant bubbles shining in gamma-ray light lie above and below the center of the Milky Way Galaxy, as seen by the Fermi satellite. (The gamma-ray and X-ray image is superimposed on a visible-light image of the inner parts of our Galaxy.) The bubbles may be evidence that the supermassive black hole at the center of our Galaxy was a quasar a few million years ago. (credit: modification of work by NASA’s Goddard Space Flight Center)
The physics required to account for the exact way in which the energy of infalling material is converted to radiation near a black hole is far more complicated than our simple discussion suggests. To understand what happens in the “rough and tumble” region around a massive black hole, astronomers and physicists must resort to computer simulations (and they require supercomputers, fast machines capable of awesome numbers of calculations per second). The details of these models are beyond the scope of our book, but they support the basic description presented here.
Radio Jets
So far, our model seems to explain the central energy source in quasars and active galaxies. But, as we have seen, there is more to quasars and other active galaxies than the point-like energy source. They can also have long jets that glow with radio waves, light, and sometimes even X-rays, and that extend far beyond the limits of the parent galaxy. Can we find a way for our black hole and its accretion disk to produce these jets of energetic particles as well?
Many different observations have now traced these jets to within 3 to 30 light-years of the parent quasar or galactic nucleus. While the black hole and accretion disk are typically smaller than 1 light-year, we nevertheless presume that if the jets come this close, they probably originate in the vicinity of the black hole. Another characteristic of the jets we need to explain is that they contain matter moving close to the speed of light.
Why are energetic electrons and other particles near a supermassive black hole ejected into jets, and often into two oppositely directed jets, rather than in all directions? Again, we must use theoretical models and supercomputer simulations of what happens when a lot of material whirls inward in a crowded black hole accretion disk. Although there is no agreement on exactly how jets form, it has become clear that any material escaping from the neighborhood of the black hole has an easier time doing so perpendicular to the disk.
In some ways, the inner regions of black hole accretion disks resemble a baby that is just learning to eat by herself. As much food as goes into the baby’s mouth can sometimes wind up being spit out in various directions. In the same way, some of the material whirling inward toward a black hole finds itself under tremendous pressure and orbiting with tremendous speed. Under such conditions, simulations show that a significant amount of material can be flung outward—not back along the disk, where more material is crowding in, but above and below the disk. If the disk is thick (as it tends to be when a lot of material falls in quickly), it can channel the outrushing material into narrow beams perpendicular to the disk (Figure 5).
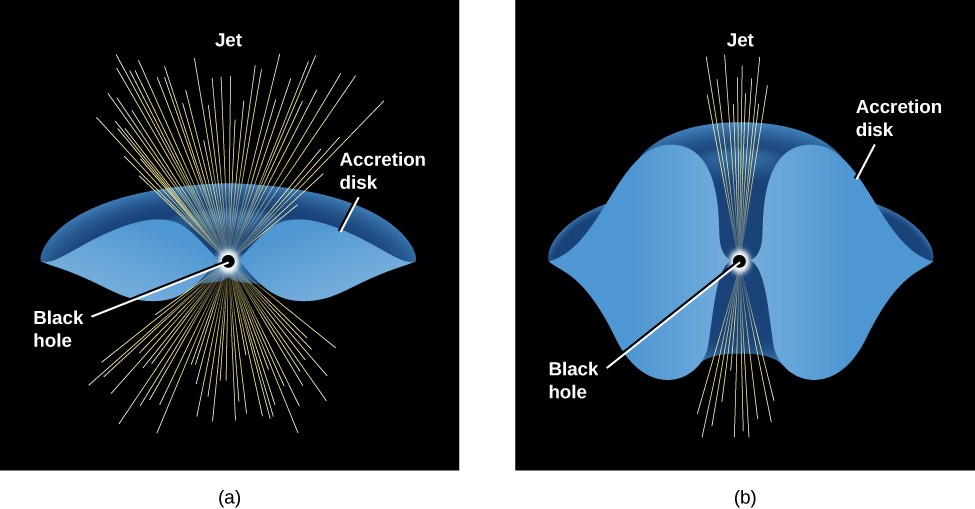
Figure 5. Models of Accretion Disks: These schematic drawings show what accretion disks might look like around large black holes for (a) a thin accretion disk and (b) a “fat” disk—the type needed to account for channeling the outflow of hot material into narrow jets oriented perpendicular to the disk.
Figure 6 shows observations of an elliptical galaxy that behaves in exactly this way. At the center of this active galaxy, there is a ring of dust and gas about 400 light-years in diameter, surrounding a 1.2-billion-MSun black hole. Radio observations show that two jets emerge in a direction perpendicular to the ring, just as the model predicts.
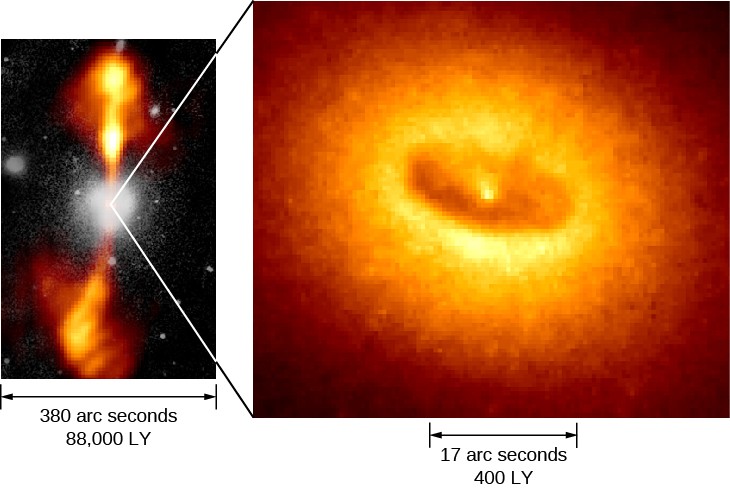
Figure 6. Jets and Disk in an Active Galaxy: The picture on the left shows the active elliptical galaxy NGC 4261, which is located in the Virgo Cluster at a distance of about 100 million light-years. The galaxy itself—the white circular region in the center—is shown the way it looks in visible light, while the jets are seen at radio wavelengths. A Hubble Space Telescope image of the central portion of the galaxy is shown on the right. It contains a ring of dust and gas about 800 light-years in diameter, surrounding a supermassive black hole. Note that the jets emerge from the galaxy in a direction perpendicular to the plane of the ring. (credit: modification of work by ESA/HST)
Quasars and the Attitudes of Astronomers
The discovery of quasars in the early 1960s was the first in a series of surprises astronomers had in store. Within another decade they would find neutron stars (in the form of pulsars), the first hints of black holes (in binary X-ray sources), and even the radio echo of the Big Bang itself. Many more new discoveries lay ahead.
As Maarten Schmidt reminisced in 1988, “This had, I believe, a profound impact on the conduct of those practicing astronomy. Before the 1960s, there was much authoritarianism in the field. New ideas expressed at meetings would be instantly judged by senior astronomers and rejected if too far out.” We saw a good example of this in the trouble Chandrasekhar had in finding acceptance for his ideas about the death of stars with cores greater than 1.4 MSun (see the feature box on Subrahmanyan Chandrasekhar in Chapter 23).
“The discoveries of the 1960s,” Schmidt continued, “were an embarrassment, in the sense that they were totally unexpected and could not be evaluated immediately. In reaction to these developments, an attitude has evolved where even outlandish ideas in astronomy are taken seriously. Given our lack of solid knowledge in extragalactic astronomy, this is probably to be preferred over authoritarianism.”[1]
That is not to say that astronomers (being human) don’t continue to have prejudices and preferences. For example, a small group of astronomers who thought that the redshifts of quasars were not connected with their distances (which was definitely a minority opinion) often felt excluded from meetings or from access to telescopes in the 1960s and 1970s. It’s not so clear that they actually were excluded, as much as that they felt the very difficult pressure of knowing that most of their colleagues strongly disagreed with them. As it turned out, the evidence—which must ultimately decide all scientific questions—was not on their side either.
But today, as better instruments bring solutions to some problems and starkly illuminate our ignorance about others, the entire field of astronomy seems more open to discussing unusual ideas. Of course, before any hypotheses become accepted, they must be tested—again and again—against the evidence that nature itself reveals. Still, the many strange proposals published about what dark matter might be (see The Evolution and Distribution of Galaxies) attest to the new openness that Schmidt described.
With this black hole model, we have come a long way toward understanding the quasars and active galaxies that seemed very mysterious only a few decades ago. As often happens in astronomy, a combination of better instruments (making better observations) and improved theoretical models enabled us to make significant progress on a puzzling aspect of the cosmos.
Key Concepts and Summary
Both active galactic nuclei and quasars derive their energy from material falling toward, and forming a hot accretion disk around, a massive black hole. This model can account for the large amount of energy emitted and for the fact that the energy is produced in a relatively small volume of space. It can also explain why jets coming from these objects are seen in two directions: those directions are perpendicular to the accretion disk.
Candela Citations
- Astronomy. Provided by: OpenStax CNX. Located at: http://cnx.org/contents/2e737be8-ea65-48c3-aa0a-9f35b4c6a966@10.1. License: CC BY: Attribution. License Terms: Download for free at http://cnx.org/contents/2e737be8-ea65-48c3-aa0a-9f35b4c6a966@10.1.
- M. Schmidt, “The Discovery of Quasars,” in Modern Cosmology in Retrospect, ed. B. Bertotti et al. (Cambridge University Press, 1990). ↵