Learning Objectives
In this module, the following topics are covered: 1) growth of electricity as a clean, versatile, switchable energy carrier; 2) components of the electricity grid – generation, delivery, use, and 3) challenges to the modern electricity grid
After reading this module, students should be able to
- outline the growth of electricity as a clean, versatile, switchable energy carrier
- understand the components of the electricity grid – generation, delivery, use
- understand the challenges to the modern electricity grid – capacity, reliability, accommodating renewables
Introduction
Over the past century and a half electricity has emerged as a popular and versatile energy carrier. Communication was an early widespread use for electricity following the introduction of the telegraph in the 1840s. In the 1870s and 1880s electric motors and lights joined the telegraph as practical electrical devices, and in the 1890s electricity distribution systems, the forerunners of today’s electricity grid, began to appear. The telegraph became wireless with the invention of radio, demonstrated in the laboratory in the 1880s and for transatlantic communication in 1901. Today, electricity is exploited not only for its diverse end uses such as lighting, motion, refrigeration, communication and computation, but also as a primary carrier of energy. Electricity is one of two backbones of the modern energy system (liquid transportation fuels are the other), carrying high density energy over short and long distances for diverse uses. In 2009, electricity consumed the largest share of the United States’ primary energy, 38 percent, with transportation a close second at 37 percent (EIA Annual Energy Review, 2009). These two sectors also accounted for the largest shares of U.S. carbon emissions, 38 percent for electricity and 33 percent for transportation (EIA Annual Energy Review, 2009). Figure United States Electricity Net Generation Since 1949 and Uses shows the growth of electricity as an energy carrier since 1949 and the growing range of its uses.
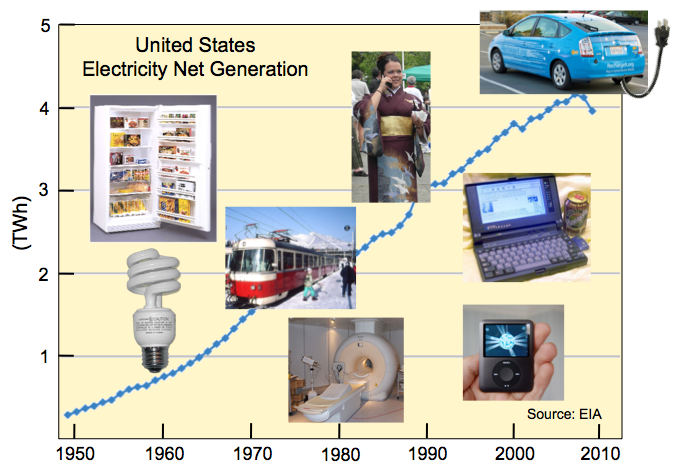
United States Electricity Net Generation Since 1949 and Uses The growth of United States electricity generation since 1949 and some of its uses. Source: G. Crabtree using data from EIA Annual Energy Review 2009, Table 8.2a, p 230.; Felix O, U.S. CPSC, Joe Mabel,Marcin Wichary, Samboy, Andrew, Jan Ainali, Lovelac7
Figure Electricity Energy Chain shows the electricity energy chain from generation to use. By far most electricity is generated by combustion of fossil fuels to turn steam or gas turbines. This is the least efficient step in the energy chain, converting only 36 percent of the chemical energy in the fuel to electric energy, when averaged over the present gas and coal generation mix. It also produces all the carbon emissions of the electricity chain. Beyond production, electricity is a remarkably clean and efficient carrier. Conversion from rotary motion of the turbine and generator to electricity, the delivery of electricity through the power grid, and the conversion to motion in motors for use in industry, transportation and refrigeration can be more than 90 percent efficient. None of these steps produces greenhouse gas emissions. It is the post-production versatility, cleanliness, and efficiency of electricity that make it a prime energy carrier for the future. Electricity generation, based on relatively plentiful domestic coal and gas, is free of immediate fuel security concerns. The advent of electric cars promises to increase electricity demand and reduce dependency on foreign oil, while the growth of renewable wind and solar generation reduces carbon emissions. The primary sustainability challenges for electricity as an energy carrier are at the production step: efficiency and emission of carbon dioxide and toxins.
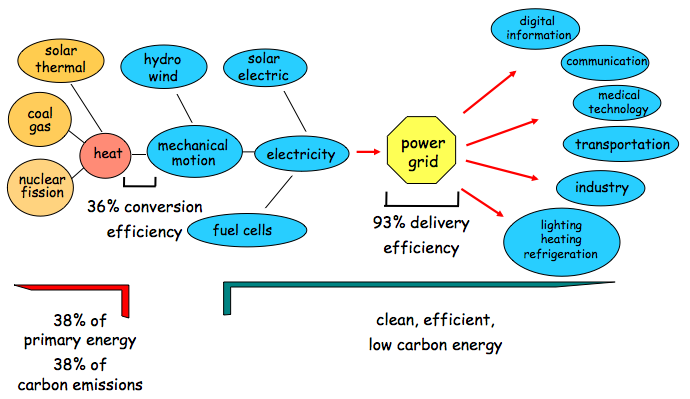
Electricity Energy Chain Graph shows the electricity energy chain from generation to use. Source: G. Crabtree
The Electricity Grid: Capacity and Reliability
Beyond production, electricity faces challenges of capacity, reliability, and implementing storage and transmission required to accommodate the remoteness and variability of renewables. The largest capacity challenges are in urban areas, where 79 percent of the United States and 50 percent of the world population live. The high population density of urban areas requires a correspondingly high energy and electric power density. In the United States, 33 percent of electric power is used in the top 22 metro areas, and electricity demand is projected to grow 31 percent by 2035 (Annual Energy Outlook, 2011). This creates an “urban power bottleneck” where underground cables become saturated, hampering economic growth and the efficiencies of scale in transportation, energy use and greenhouse gas emission that come with high population density (Owen, 2009). Saturation of existing cable infrastructure requires installation of substantial new capacity, an expensive proposition for digging new underground cable tunnels.
Superconducting underground cables with five times the power delivery capacity of conventional copper offer an innovative alternative (see Figure Superconducting Underground Cables). Unlike conventional cables, superconducting cables emit no heat or electromagnetic radiation, eliminating interference with other underground energy and communication infrastructure. Replacing conventional with superconducting cables in urban areas dramatically increases capacity while avoiding the construction expense of additional underground infrastructure.
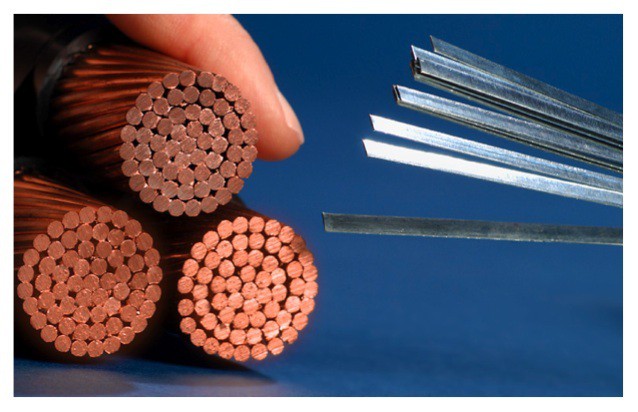
Superconducting Underground Cables The superconducting wires on the right carry the same current as the conventional copper wires on the left. Superconducting cable wound from these wires carries up to five times the current of conventional copper cables. Source: Courtesy, American Superconductor Corporation
The reliability of the electricity grid presents a second challenge. The United States’ grid has grown continuously from origins in the early 20th Century; much of its infrastructure is based on technology and design philosophy dating from the 1950s and 1960s, when the major challenge was extending electrification to new rural and urban areas. Outside urban areas, the grid is mainly above ground, exposing it to weather and temperature extremes that cause most power outages. The response to outages is frustratingly slow and traditional – utilities are often first alerted to outages by telephoned customer complaints, and response requires sending crews to identify and repair damage, much the same as we did 50 years ago. The United States’ grid reliability is significantly lower than for newer grids in Europe and Japan, where the typical customer experiences ten to 20 times less outage time than in the United States. Reliability is especially important in the digital age, when an interruption of even a fraction of a cycle can shut down a digitally controlled data center or fabrication line, requiring hours or days to restart.
Reliability issues can be addressed by implementing a smart grid with two-way communication between utility companies and customers that continuously monitors power delivery, the operational state of the delivery system, and implements demand response measures adjusting power delivered to individual customers in accordance with a previously established unique customer protocol. Such a system requires installing digital sensors that monitor power flows in the delivery system, digital decision and control technology and digital communication capability like that already standard for communication via the Internet. For customers with on-site solar generation capability, the smart grid would monitor and control selling excess power from the customer to the utility.
Figure Smart Grid illustrates the two-way communication features of the smart grid. The conventional grid in the upper panel sends power one way, from the generating station to the customer, recording how much power leaves the generator and arrives at the customer. In the smart grid, the power flow is continuously monitored, not only at the generator and the customer, but also at each connection point in between. Information on the real time power flow is sent over the Internet or another special network to the utility and to the customer, allowing real time decisions on adding generation to meet changes in load, opening circuit breakers to reroute power in case of an outage, reducing power delivered to the customer during peak periods to avoid outages (often called “demand response”), and tracking reverse power flows for customers with their own solar or other generation capacity. The conventional power grid was designed in the middle of the last century to meet the simple need of delivering power in one direction. Incorporating modern Internet-style communications and control features could bring the electricity grid to a qualitatively new level of capability and performance required to accommodate local generation and deliver higher reliability.
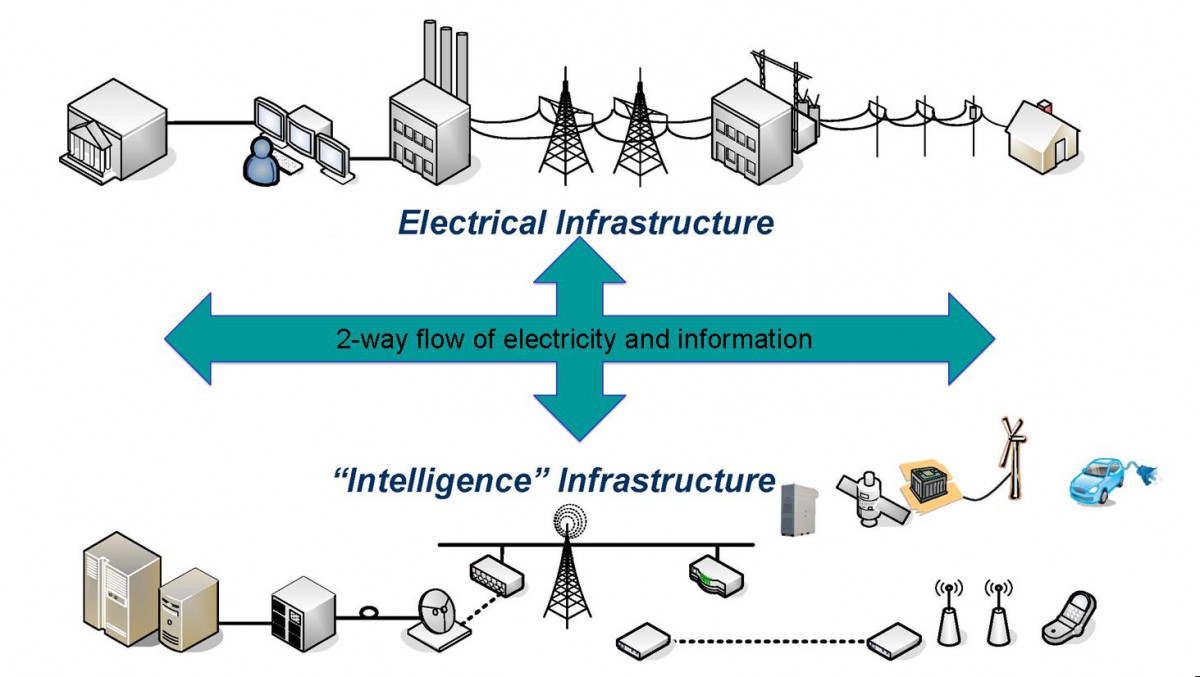
Smart Grid The addition of real-time monitoring and communicating capability like that used on the Internet would add ‘smart’ operation of the electricity grid. Source: National Institute of Standards and Technology
Smart components incorporated throughout the grid would be able to detect overload currents and open breakers to interrupt them quickly and automatically to avoid unnecessary damage and triggering a domino effect cascade of outages over wide areas as happened in the Northeast Blackout of 2003. For maximum effectiveness, such smart systems require fast automatic response on millisecond time scales commensurate with the cycle time of the grid. Even simple digital communication meets this requirement, but many of the grid components themselves cannot respond so quickly. Conventional mechanical circuit breakers, for example, take many seconds to open and much longer to close. Such long times increase the risk of dangerous overload currents damaging the grid or propagating cascades. Along with digital communications, new breaker technology, such as that based on fast, self-healing superconducting fault current limiters, is needed to bring power grid operation into the modern era.
Integrating Renewable Electricity on the Grid
Accommodating renewable electricity generation by wind and solar plants is among the most urgent challenges facing the grid. Leadership in promoting renewable electricity has moved from the federal to the state governments, many of which have legislated Renewable Portfolio Standards (RPS) that require 20 percent of state electricity generation to be renewable by 2020. 30 states and the District of Columbia have such requirements, the most aggressive being California with 33 percent renewable electricity required by 2020 and New York with 30 percent by 2015. To put this legal requirement in perspective, wind and solar now account for about 1.6 percent of U.S. electricity production; approximately a factor of ten short of the RPS requirements. (Crabtree & Misewich, 2010).
Renewable Variability
The grid faces major challenges to accommodate the variability of wind and solar electricity. Without significant storage capacity, the grid must precisely balance generation to demand in real time. At present, the variability of demand controls the balancing process: demand varies by as much as a factor of two from night to day as people go through their daily routines. This predictable variability is accommodated by switching reserve generation sources in and out in response to demand variations. With renewable generation, variation can be up to 70 percent for solar electricity due to passing clouds and 100 percent for wind due to calm days, much larger than the variability of demand. At the present level of 1.6 percent wind and solar penetration, the relatively small variation in generation can be accommodated by switching in and out conventional resources to make up for wind and solar fluctuations. At the 20 percent penetration required by state Renewable Portfolio Standards, accommodating the variation in generation requires a significant increase in the conventional reserve capacity. At high penetration levels, each addition of wind or solar capacity requires a nearly equal addition of conventional capacity to provide generation when the renewables are quiescent. This double installation to insure reliability increases the cost of renewable electricity and reduces its effectiveness in lowering greenhouse gas emissions.
A major complication of renewable variation is its unpredictability. Unlike demand variability, which is reliably high in the afternoon and low at night, renewable generation depends on weather and does not follow any pattern. Anticipating weather-driven wind and solar generation variability requires more sophisticated forecasts with higher accuracy and greater confidence levels than are now available. Because today’s forecasts often miss the actual performance target, additional conventional reserves must be held at the ready to cover the risk of inaccuracies, adding another increase to the cost of renewable electricity.
Storing Electricity
Storage of renewable electricity offers a viable route to meeting the variable generation challenge. Grid electricity storage encompasses many more options than portable electricity storage required for electric cars. Unlike vehicle storage, grid storage can occupy a large footprint with little or no restriction on weight or volume. Grid storage can be housed in a controlled environment, eliminating large temperature and humidity variations that affect performance. Grid storage must have much higher capacity than vehicle storage, of order 150 MWh for a wind farm versus 20-50 kWh for a vehicle. Because of these differences, the research strategy for grid and vehicle energy storage is very different. To date, much more attention has been paid to meeting vehicle electricity storage requirements than grid storage requirements.
There are many options for grid storage. Pumped hydroelectric storage, illustrated in Figure Pumped Hydroelectric Storage, is an established technology appropriate for regions with high and low elevation water resources. Compressed Air Energy Storage (CAES) is a compressed air equivalent of pumped hydro that uses excess electricity to pump air under pressure into underground geologic formations for later release to drive generators. This option has been demonstrated in Huntorf, Germany and in Mcintosh, Alabama. High temperature sodium-sulfur batteries operating at 300 °C have high energy density, projected long cycle life, and high round trip efficiency; they are the most mature of the battery technologies suggested for the grid. Flow batteries are an attractive and relatively unexplored option, where energy is stored in the high charge state of a liquid electrolyte and removed by electrochemical conversion to a low charge state. Each flow battery requires an electrolyte with a high and low charge state and chemical reaction that takes one into the other. There are many such electrolytes and chemical reactions, of which only a few have been explored, leaving a host of promising opportunities for the future. The energy storage capacity depends only on the size of the storage tank, which can be designed fully independently of the power capacity that depends on the size of the electrochemical reactor. Sodium sulfur and flow batteries store electric charge and can be used at any place in the electricity grid. In contrast, thermal storage applies only to concentrating solar power technologies, where mirrors focus solar radiation to heat a working fluid that drives a conventional turbine and generator. In these systems, heat energy can be stored as a molten salt in a highly insulated enclosure for hours or days, allowing solar electricity to be generated on demand after sunset or on cloudy days. All of these options are promising and require research and development to explore innovations, performance and cost limits.
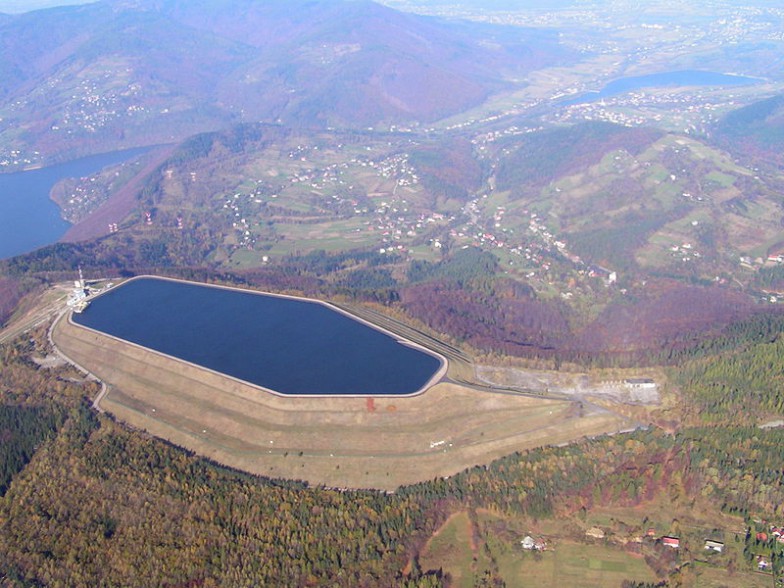
Pumped Hydroelectric Storage Upper storage reservoir for pumped hydroelectric storage, an established technology for storing large amounts of grid electricity. Source: Ongrys via Wikimedia Commons
How to Transmit Electricity Over Long Distances
The final challenge for accommodating renewables is long distance transmission. As Figure Renewable Resource Location vs. Demand Location shows, the largest wind resources, located at mid-continent, and the largest solar resources, in the southwest, are far from the population centers east of Mississippi and on the West Coast. If these resources are to be used, higher capacity long distance transmission must be developed to bring the renewable electricity to market. Although such long distance delivery is possible where special high voltage transmission lines have been located, the capacity and number of such lines is limited. The situation is much like automobile transportation before the interstate highway system was built in the 1950s. It was possible to drive coast to coast, but the driving time was long and uncertain and the route indirect. To use renewable electricity resources effectively, we must create a kind of interstate highway system for electricity.
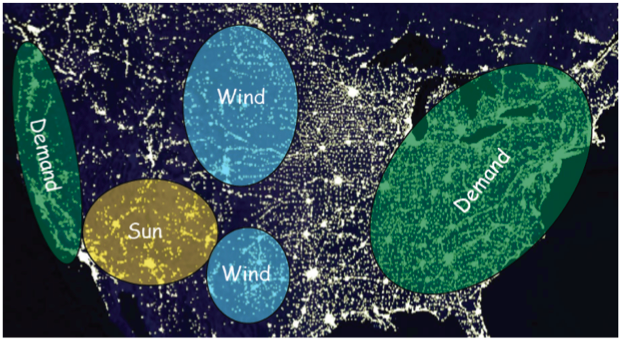
Renewable Resource Location vs. Demand Location Wind and solar electricity resources are located far from population centers, requiring a dramatic improvement in long-distance electricity transmission – an “interstate highway system for electricity.” Source: Integrating Renewable Electricity on the Grid, Report of the Panel on Pubic Affairs, American Physical Society (2010).
Summary
Electricity and liquid petroleum are the two primary energy carriers in the United States, and in the world. Once produced, electricity is clean and versatile making it an appealing energy carrier for the future. The challenges facing the electricity grid are capacity, reliability, and accommodating renewable sources such as solar and wind whose output is variable and whose location is remote from population centers. Electricity storage and long distance transmission are needed to accommodate these renewable resources.
Review Questions
Electricity is the fastest growing energy carrier in the world, trailed by liquid fuels for transportation. Why is electricity more appealing than liquid fuels?
A primary challenge for the electricity grid is capacity to handle the “urban power bottleneck” in cities and suburbs. How can superconducting cables address urban capacity issues?
Renewable wind and solar electricity is plentiful in the United States, but they are located remotely from high population centers and their output is variable in time. How can these two issues be addressed?
References
Crabtree, G. & Misewich, J. (Co-Chairs). (2010). Integrating Renewable Electricity on the Grid, American Physical Society. American Physical Society, Washington D.C. Retrieved August 12, 2011 from http://www.aps.org/policy/reports/popa-reports/upload/integratingelec.pdf
Owen, D. (2009). Green Metropolis: Why Living Smaller, Living Closer, And Driving Less Are the Keys to Sustainability. New York: Riverhead Books.
U.S. Energy Information Administration. (2010). Annual Energy Review 2009. Retrieved August 12, 2011 from http://www.eia.gov/totalenergy/data/annual/pdf/aer.pdf
Glossary
- efficiency
- The fraction of energy at the input that is delivered to the output of a device. Electric motors can convert incoming electricity to rotary motion at more than 90 percent efficiency, while gasoline engines convert only about 25 percent of the chemical energy of the fuel to motion of the wheels.
- electricity grid
- The network of wires and transformers that delivers electric power from generation stations such as those powered by coal, natural gas, hydroelectricity, sunlight or wind to end uses such as lighting, transportation, refrigeration, computation or communication. The electricity grid is conventionally divided into higher voltage transmission lines for long distances, lower voltage distribution lines for short distances and transformers in substations for converting the voltage between the two categories.
- kWh and MWh
- Units of energy used in power engineering. kWh is one kilowatt of power delivered for one hour, MWh is one megawatt of power delivered for one hour.
- primary energy
- The energy embodied in natural resources prior to undergoing any human-made conversions or transformations. Examples include the chemical energy in coal or the sunlight falling on a solar cell before it is converted to electricity, the nuclear energy in the fuel of a nuclear reactor, or the kinetic energy of wind before it turns the blades of a turbine.
- renewable generation variability
- The variation of the output of a solar or wind plant depending on weather. Solar plants often produce only 15-20 percent of their maximum output (also called installed capacity) because the sun shines only during the day and passing clouds can obscure it; wind plants produce 20-40 percent of their maximum capacity because the wind speed varies with weather conditions, often becoming calm for days at a time.
- smart grid
- The addition of sensors to monitor power flow and two-way communication to transmit the power flow information to the utility and the customer in real time. The addition of sensors and communication to the grid enables several new operating modes: the customer decide in real time to curtail his electricity use during peak times when rates are high (known as demand-response), the utility can identify precisely the time and place of power flow failures due to weather or other events, and the grid can be equipped with automatic circuit breakers (known as fault current limiters) and other protection devices that respond immediately to power flow failures, limiting damage to the grid and the risk of triggering a cascade of failures.
- superconducting cable
- An underground cable made of superconductor, which loses all resistance to electric current at low temperature. Superconducting cables made of second-generation coated conductors based on the copper oxide family of superconductors discovered in 1986 are now entering the grid. Because superconductors conduct electricity without producing heat, they can carry up to five times more power than conventional copper cables in the same cross-sectional area.
Candela Citations
- Sustainability: A Comprehensive Foundation. Authored by: Tom Theis and Jonathan Tomkin, Editors.. Provided by: OpenStax CNX. Located at: http://cnx.org/contents/1741effd-9cda-4b2b-a91e-003e6f587263@44.1. License: CC BY: Attribution. License Terms: Download for free at http://cnx.org/contents/1741effd-9cda-4b2b-a91e-003e6f587263@44.1