Learning Objectives
By the end of this section, you will be able to:
- Describe how the observations of protoplanetary disks provides evidence for the existence of other planetary systems
- Explain the two primary methods for detection of exoplanets
- Compare the main characteristics of other planetary systems with the features of the solar system
Until the middle 1990s, the practical study of the origin of planets focused on our single known example—the solar system. Although there had been a great deal of speculation about planets circling other stars, none had actually been detected. Logically enough, in the absence of data, most scientists assumed that our own system was likely to be typical. They were in for a big surprise.
Discovery of Other Planetary Systems
In The Birth of Stars and the Discovery of Planets outside the Solar System, we discuss the formation of stars and planets in some detail. Stars like our Sun are formed when dense regions in a molecular cloud (made of gas and dust) feel an extra gravitational force and begin to collapse. This is a runaway process: as the cloud collapses, the gravitational force gets stronger, concentrating material into a protostar. Roughly half of the time, the protostar will fragment or be gravitationally bound to other protostars, forming a binary or multiple star system—stars that are gravitationally bound and orbit each other. The rest of the time, the protostar collapses in isolation, as was the case for our Sun. In all cases, as we saw, conservation of angular momentum results in a spin-up of the collapsing protostar, with surrounding material flattened into a disk. Today, this kind of structure can actually be observed. The Hubble Space Telescope, as well as powerful new ground-based telescopes, enable astronomers to study directly the nearest of these circumstellar disks in regions of space where stars are being born today, such as the Orion Nebula ([link]) or the Taurus star-forming region.
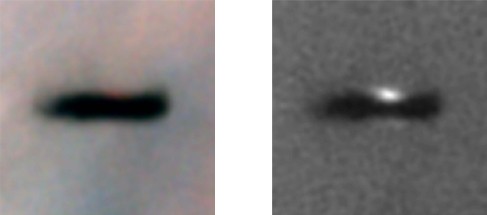
Many of the circumstellar disks we have discovered show internal structure. The disks appear to be donut-shaped, with gaps close to the star. Such gaps indicate that the gas and dust in the disk have already collapsed to form large planets ([link]). The newly born protoplanets are too small and faint to be seen directly, but the depletion of raw materials in the gaps hints at the presence of something invisible in the inner part of the circumstellar disk—and that something is almost certainly one or more planets. Theoretical models of planet formation, like the one seen at right in [link], have long supported the idea that planets would clear gaps as they form in disks.
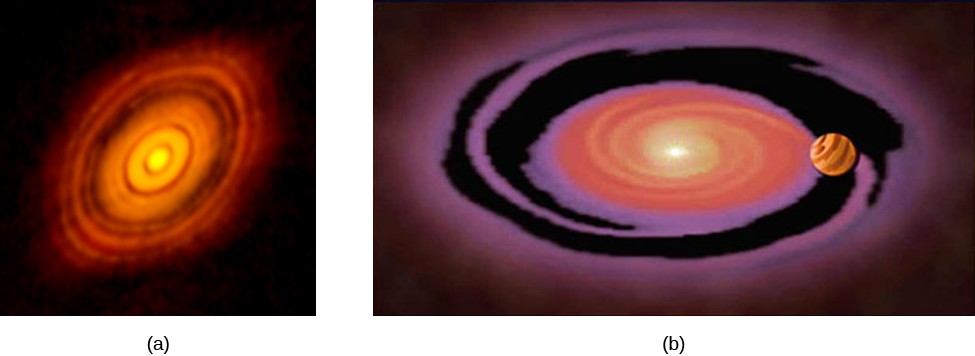
Our figure shows HL Tau, a one-million-year-old “newborn” star in the Taurus star-forming region. The star is embedded in a shroud of dust and gas that obscures our visible-light view of a circumstellar disk around the star. In 2014 astronomers obtained a dramatic view of the HL Tau circumstellar disk using millimeter waves, which pierce the cocoon of dust around the star, showing dust lanes being carved out by several newly formed protoplanets. As the mass of the protoplanets increases, they travel in their orbits at speeds that are faster than the dust and gas in the circumstellar disk. As the protoplanets plow through the disk, their gravitational reach begins to exceed their cross-sectional area, and they become very efficient at sweeping up material and growing until they clear a gap in the disk. The image of [link] shows us that a number of protoplanets are forming in the disk and that they were able to form faster than our earlier ideas had suggested—all in the first million years of star formation.
For an explanation of ALMA’s ground-breaking observations of HL Tau and what they reveal about plant formation, watch this videocast from the European Southern Observatory.
Discovering Exoplanets
You might think that with the advanced telescopes and detectors astronomers have today, they could directly image planets around nearby stars (which we call exoplanets). This has proved extremely difficult, however, not only because the exoplanets are faint, but also because they are generally lost in the brilliant glare of the star they orbit. As we discuss in more detail in The Birth of Stars and the Discovery of Planets outside the Solar System, the detection techniques that work best are indirect: they observe the effects of the planet on the star it orbits, rather than seeing the planet itself.
The first technique that yielded many planet detections is very high-resolution stellar spectroscopy. The Doppler effect lets astronomers measure the star’s radial velocity: that is, the speed of the star, toward us or away from us, relative to the observer. If there is a massive planet in orbit around the star, the gravity of the planet causes the star to wobble, changing its radial velocity by a small but detectable amount. The distance of the star does not matter, as long as it is bright enough for us to take very high quality spectra.
Measurements of the variation in the star’s radial velocity as the planet goes around the star can tell us the mass and orbital period of the planet. If there are several planets present, their effects on the radial velocity can be disentangled, so the entire planetary system can be deciphered—as long as the planets are massive enough to produce a measureable Doppler effect. This detection technique is most sensitive to large planets orbiting close to the star, since these produce the greatest wobble in their stars. It has been used on large ground-based telescopes to detect hundreds of planets, including one around Proxima Centauri, the nearest star to the Sun.
The second indirect technique is based on the slight dimming of a star when one of its planets transits, or crosses over the face of the star, as seen from Earth. Astronomers do not see the planet, but only detect its presence from careful measurements of a change in the brightness of the star over long periods of time. If the slight dips in brightness repeat at regular intervals, we can determine the orbital period of the planet. From the amount of starlight obscured, we can measure the planet’s size.
While some transits have been measured from Earth, large-scale application of this transit technique requires a telescope in space, above the atmosphere and its distortions of the star images. It has been most successfully applied from the NASA Kepler space observatory, which was built for the sole purpose of “staring” for 5 years at a single part of the sky, continuously monitoring the light from more than 150,000 stars. The primary goal of Kepler was to determine the frequency of occurrence of exoplanets of different sizes around different classes of stars. Like the Doppler technique, the transit observations favor discovery of large planets and short-period orbits.
Recent detection of exoplanets using both the Doppler and transit techniques has been incredibly successful. Within two decades, we went from no knowledge of other planetary systems to a catalog of thousands of exoplanets. Most of the exoplanets found so far are more massive than or larger in size than Earth. It is not that Earth analogs do not exist. Rather, the shortage of small rocky planets is an observational bias: smaller planets are more difficult to detect.
Analyses of the data to correct for such biases or selection effects indicate that small planets (like the terrestrial planets in our system) are actually much more common than giant planets. Also relatively common are “super Earths,” planets with two to ten times the mass of our planet ([link]). We don’t have any of these in our solar system, but nature seems to have no trouble making them elsewhere. Overall, the Kepler data suggest that approximately one quarter of stars have exoplanet systems, implying the existence of at least 50 billion planets in our Galaxy alone.
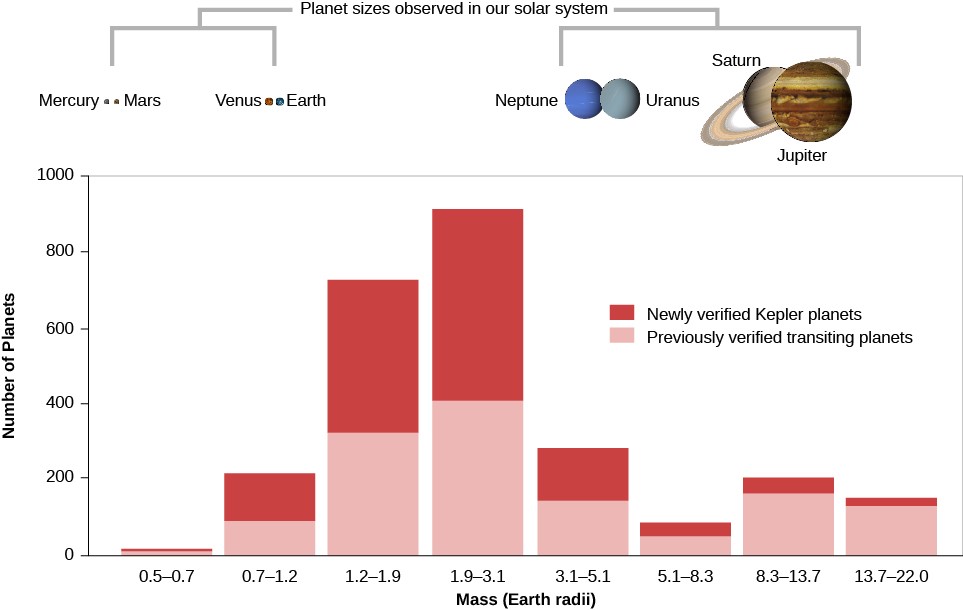
The Configurations of Other Planetary Systems
Let’s look more closely at the progress in the detection of exoplanets. [link] shows the planets that were discovered each year by the two techniques we discussed. In the early years of exoplanet discovery, most of the planets were similar in mass to Jupiter. This is because, as mentioned above, the most massive planets were easiest to detect. In more recent years, planets smaller than Neptune and even close to the size of Earth have been detected.
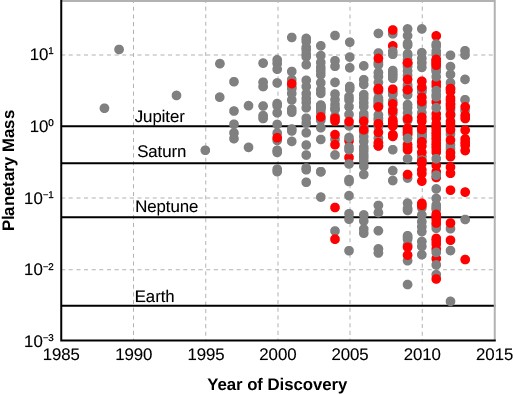
We also know that many exoplanets are in multiplanet systems. This is one characteristic that our solar system shares with exosystems. Looking back at [link] and seeing how such large disks can give rise to more than one center of condensation, it is not too surprising that multiplanet systems are a typical outcome of planet formation. Astronomers have tried to measure whether multiple planet systems all lie in the same plane using astrometry. This is a difficult measurement to make with current technology, but it is an important measurement that could help us understand the origin and evolution of planetary systems.
Comparison between Theory and Data
Many of the planetary systems discovered so far do not resemble our own solar system. Consequently, we have had to reassess some aspects of the “standard models” for the formation of planetary systems. Science sometimes works in this way, with new data contradicting our expectations. The press often talks about a scientist making experiments to “confirm” a theory. Indeed, it is comforting when new data support a hypothesis or theory and increase our confidence in an earlier result. But the most exciting and productive moments in science often come when new data don’t support existing theories, forcing scientists to rethink their position and develop new and deeper insights into the way nature works.
Nothing about the new planetary systems contradicts the basic idea that planets form from the aggregation (clumping) of material within circumstellar disks. However, the existence of “hot Jupiters”—planets of jovian mass that are closer to their stars than the orbit of Mercury—poses the biggest problem. As far as we know, a giant planet cannot be formed without the condensation of water ice, and water ice is not stable so close to the heat of a star. It seems likely that all the giant planets, “hot” or “normal,” formed at a distance of several astronomical units from the star, but we now see that they did not necessarily stay there. This discovery has led to a revision in our understanding of planet formation that now includes “planet migrations” within the protoplanetary disk, or later gravitational encounters between sibling planets that scatter one of the planets inward.
Many exoplanets have large orbital eccentricity (recall this means the orbits are not circular). High eccentricities were not expected for planets that form in a disk. This discovery provides further support for the scattering of planets when they interact gravitationally. When planets change each other’s motions, their orbits could become much more eccentric than the ones with which they began.
There are several suggestions for ways migration might have occurred. Most involve interactions between the giant planets and the remnant material in the circumstellar disk from which they formed. These interactions would have taken place when the system was very young, while material still remained in the disk. In such cases, the planet travels at a faster velocity than the gas and dust and feels a kind of “headwind” (or friction) that causes it to lose energy and spiral inward. It is still unclear how the spiraling planet stops before it plunges into the star. Our best guess is that this plunge into the star is the fate for many protoplanets; however, clearly some migrating planets can stop their inward motions and escape this destruction, since we find hot Jupiters in many mature planetary systems.
Key Concepts and Summary
The first planet circling a distant solar-type star was announced in 1995. Twenty years later, thousands of exoplanets have been identified, including planets with sizes and masses between Earth’s and Neptune’s, which we don’t have in our own solar system. A few percent of exoplanet systems have “hot Jupiters,” massive planets that orbit close to their stars, and many exoplanets are also in eccentric orbits. These two characteristics are fundamentally different from the attributes of gas giant planets in our own solar system and suggest that giant planets can migrate inward from their place of formation where it is cold enough for ice to form. Current data indicate that small (terrestrial type) rocky planets are common in our Galaxy; indeed, there must be tens of billions of such earthlike planets.
Glossary
- exoplanet
- a planet orbiting a star other than our Sun
Candela Citations
- Astronomy. Provided by: OpenStax CNX. Located at: http://cnx.org/contents/2e737be8-ea65-48c3-aa0a-9f35b4c6a966@10.1. License: CC BY: Attribution. License Terms: Download for free at http://cnx.org/contents/2e737be8-ea65-48c3-aa0a-9f35b4c6a966@10.1.