Learning Outcomes
- Identify the structure and function of the sensory systems
We experience reality through our senses. Senses are the physiological methods of perception, so a sense is a faculty by which outside stimuli are perceived. The senses and their operation, classification, and theory are overlapping topics studied by a variety of fields. Many neurologists disagree about how many senses there actually are due to a broad interpretation of the definition of a sense. Our senses are split into two different groups. Our exteroceptors detect stimulation from the outsides of our body: this includes smell, taste, and equilibrium. The interoceptors receive stimulation from the inside of our bodies: this includes blood pressure dropping, changes in the glucose, and pH levels. Children are generally taught that there are five senses (sight, hearing, touch, smell, taste). However, it is generally agreed that there are at least seven different senses in humans, and a minimum of two more observed in other organisms. Sense can also differ from one person to the next. Take taste for an example: what may taste great to one person will taste awful to someone else. This has to do with how the brain interprets the stimuli that are received.
Chemoreception
The senses of gustation (taste) and olfaction (smell) fall under the category of chemoreception. Specialized cells act as receptors for certain chemical compounds. As these compounds react with the receptors, an impulse is sent to the brain and is registered as a certain taste or smell. Gustation and olfaction are chemical senses because the receptors they contain are sensitive to the molecules in the food we eat, along with the air we breathe.
Gustatory System
In humans, the sense of taste is transduced by taste buds and is conveyed via three of the twelve cranial nerves. Cranial nerve VII, the facial nerve, carries taste sensations from the anterior two thirds of the tongue (excluding the circumvallate papillae, see lingual papilla) and soft palate. Cranial nerve IX the glossopharyngeal nerve carries taste sensations from the posterior one third of the tongue (including the circumvallate papillae). Also a branch of the vagus nerve carries some taste sensations from the back of the oral cavity (i.e., pharynx and epiglottis). Information from these cranial nerves is processed by the gustatory system. Though there are small differences in sensation, which can be measured with highly specific instruments, all taste buds can respond to all types of taste. Sensitivity to all tastes is distributed across the whole tongue and indeed to other regions of the mouth where there are taste buds (epiglottis, soft palate).
Papilla
Papilla are specialized epithelial cells. There are four types of papillae: filiform (thread-shape), fungiform (mushroom-shape), foliate (leaf-shape), and circumvallate (ringed-circle). All papillae except the filiform have taste buds on their surface. Some act directly by ion channels, others act indirectly.
- Fungiform papillae: as the name suggests, are slightly mushroom shaped if looked at in section. These are present mostly at the apex (tip) of the tongue.
- Filiform papillae: these are thin, longer papillae that don’t contain taste buds but are the most numerous. These papillae are mechanical and not involved in gustation.
- Foliate papillae: these are ridges and grooves towards the posterior part of the tongue.
- Circumvallate papillae: there are only about 3–14 of these papillae on most people and they are present at the back of the oral part of the tongue. They are arranged in a circular-shaped row just in front of the sulcus terminalis of the tongue.
Olfactory System
Olfaction is the sense of smell. In humans the sense of smell is received in nasopharynx. Airborne molecules go into solution on moist epithelial surface of nasal passage. An olfactory receptors neuron sends an impulse via Cranial nerve I the olfactory nerve. Although 80–90 percent of what we think is “taste” actually is due to smell. This is why when we have a head cold or stuffed up nose we have a harder time tasting our foods.
Receptors
Humans have 347 functional odor receptor genes; the other genes have nonsense mutations. This number was determined by analyzing the genome in the Human Genome Project; the number may vary among ethnic groups, and does vary among individuals. For example, not all people can smell androstenone, a component of male sweat.
Each olfactory receptor neuron in the nose expresses only one functional odor receptor. Odor receptor nerve cells may function like a key-lock system: if the odor molecules can fit into the lock the nerve cell will respond. According to shape theory, each receptor detects a feature of the odor molecule. Weak-shape theory, known as odotope theory, suggests that different receptors detect only small pieces of molecules, and these minimal inputs are combined to create a larger olfactory perception (similar to the way visual perception is built up of smaller, information-poor sensations, combined and refined to create a detailed overall perception). An alternative theory, the vibration theory proposed by Luca Turin[1], posits that odor receptors detect the frequencies of vibrations of odor molecules in the infrared range by electron tunneling. However, the behavioral predictions of this theory have been found lacking[2].
An olfactory receptor neuron, also called an olfactory sensory neuron, is the primary transduction cell in the olfactory system. Humans have about 40 million olfactory receptor neurons. In vertebrates, olfactory receptor neurons reside on the olfactory epithelium in the nasal cavity. These cells are bipolar neurons with a dendrite facing the interior space of the nasal cavity and an axon that travels along the olfactory nerve to the olfactory bulb.
Many tiny hair-like cilia protrude from the olfactory receptor cell’s dendrite and into the mucus covering the surface of the olfactory epithelium. These cilia contain olfactory receptors, a type of G protein-coupled receptor. Each olfactory receptor cell contains only one type of olfactory receptor, but many separate olfactory receptor cells contain the same type of olfactory receptor. The axons of olfactory receptor cells of the same type converge to form glomeruli in the olfactory bulb.
Olfactory receptors can bind to a variety of odor molecules. The activated olfactory receptor in turn activates the intracellular G-protein GOLF, and adenylate cyclase and production of Cyclic AMP opens ion channels in the cell membrane, resulting in an influx of sodium and calcium ions into the cell. This influx of positive ions causes the neuron to depolarize, generating an action potential.
Individual olfactory receptor neurons are replaced approximately every 40 days by neural stem cells residing in the olfactory epithelium. The regeneration of olfactory receptor cells, as one of the only few instances of adult neurogenesis in the central nervous system, has raised considerable interest in dissecting the pathways for neural development and differentiation in adult organisms.
In the Brain
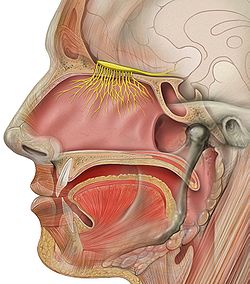
Figure 1. The Olfactory Nerve leading to the brain.
The axons from all the thousands of cells expressing the same odor receptor converge in the olfactory bulb (Figure 1). Mitral cells in the olfactory bulb send the information about the individual features to other parts of the olfactory system in the brain, which puts together the features into a representation of the odor. Since most odor molecules have many individual features, the combination of features gives the olfactory system a broad range of odors that it can detect.
Odor information is easily stored in long term memory and has strong connections to emotional memory. This is possibly due to the olfactory system’s close anatomical ties to the limbic system and hippocampus, areas of the brain that have long been known to be involved in emotion and place memory, respectively.
Pheromonal Olfaction
Some pheromones are detected by the olfactory system, although in many vertebrates pheromones are also detected by the vomeronasal organ, located in the vomer, between the nose and the mouth. Snakes use it to smell prey, sticking their tongue out and touching it to the organ. Some mammals make a face called flehmen to direct air to this organ. In humans, it is unknown whether or not pheromones exist.
Olfaction and Gustation
Olfaction, taste and trigeminal receptors together contribute to flavor. It should be emphasized that there are no more than 5 distinctive tastes: salty, sour, sweet, bitter, and umami. The 10,000 different scents which humans usually recognize as “tastes” are often lost or severely diminished with the loss of olfaction. This is the reason why food has little flavor when your nose is blocked, as from a cold.
The key nutrition players in our taste is the olfactory function, 80–90 percent of what we consider taste is dependent on our senses of smell. With aging our olfactory function declines. In the elderly careful monitoring of appetite is necessary due to the alterations in the olfactory function.
The Sense of Vision
Vision needs to have the work of both the eyes and the brain to process any information. The majority of the stimuli is done in the eyes and then the information is sent to the brain by the way of nerve impulses. At least one-third of the information of what the eye sees is processed in the cerebral cortex of the brain.
Anatomy of the Eye
The human eye is a elongated ball about 1-inch (2.5 cm) in diameter and is protected by a bony socket in the skull. The eye has three layers or coats that make up the exterior wall of the eyeball, which are the sclera, choroid, and retina.
Sclera
The outer layer of the eye is the sclera, which is a tough white fibrous layer that maintains, protects and supports the shape of the eye. The front of the sclera is transparent and is called the cornea. The cornea refracts light rays and acts like the outer window of the eye.
Choroid
The middle thin layer of the eye is the choroid, also known as the choroidea or choroid coat, it is the vascular layer of the eye lying between the retina and the sclera. The choroid provides oxygen and nourishment to the outer layers of the retina. It also contains a nonreflective pigment that acts as a light shield and prevents light from scattering. Light enters the front of the eye through a hole in the choroid coat called the pupil. The iris contracts and dilates to compensate for the changes in light intensity. If the light is bright the iris then contracts making the pupil smaller, and if the light is dim, the iris dilates making the pupil bigger. Just posterior to the iris is the lens, which is composed mainly of proteins called crystallins. The lens is attached by the zonules to the ciliary body that contains the ciliary muscles that control the shape of the lens for accommodation. Along with the ciliary body and iris, the choroid forms the uveal tract. The uvea is the middle of the three concentric layers that make up an eye. The name is possibly a reference to its almost black color, wrinkled appearance and grape-like size and shape when stripped intact from a cadaveric eye.
Eye Movement
The visual system in the brain is too slow to process that information if the images are slipping across the retina at more than a few degrees per second, thus, for humans to be able to see while moving, the brain must compensate for the motion of the head by turning the eyes. To get a clear view of the world, the brain must turn the eyes so that the image of the object of regard falls on the fovea. Eye movements are thus very important for visual perception, and any failure to make them correctly can lead to serious visual disabilities. Having two eyes is an added complication, because the brain must point both of them accurately enough that the object of regard falls on corresponding points of the two retinas; otherwise, double vision would occur. The movements of different body parts are controlled by striated muscles acting around joints. The movements of the eye are no exception, but they have special advantages not shared by skeletal muscles and joints, and so are considerably different.
Try This Experiment
Hold your hand up, about one foot (30 cm) in front of your nose. Keep your head still, and shake your hand from side to side, slowly at first, and then faster and faster. At first you will be able to see your fingers quite clearly. But as the frequency of shaking passes about one hertz, the fingers will become a blur. Now, keep your hand still, and shake your head (up and down or left and right). No matter how fast you shake your head, the image of your fingers remains clear. This demonstrates that the brain can move the eyes opposite to head motion much better than it can follow, or pursue, a hand movement. When your pursuit system fails to keep up with the moving hand, images slip on the retina and you see a blurred hand.
Depth Perception
Depth perception is the visual ability to perceive the world in three dimensions. It is a trait common to many higher animals. Depth perception allows the beholder to accurately gauge the distance to an object. Depth perception is often confused with binocular vision, also known as Stereopsis. Depth perception does rely on binocular vision, but it also uses many other monocular cues.
The Senses Of Hearing
The ear is the sense organ that collects and detects sound waves and plays a major role in the sense of balance and body position. The sensory receptors for both hearing and equilibrium are mechanoreceptors found in the inner ear; these receptors are hair cells that have stereocilia (long microvilli) that are extremely sensitive to mechanical stimulations.
Anatomy of the Ear
The ear has three divisions: the outer ear, the middle ear, and the inner ear (Figure 3).
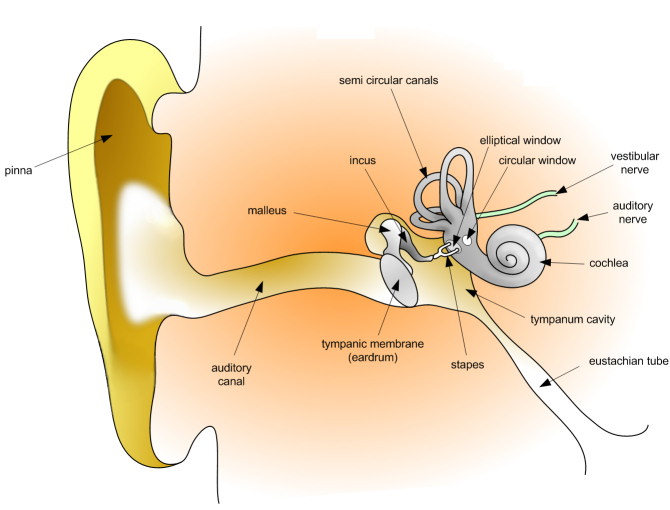
Figure 3. Anatomy of the human ear.
Outer Ear: Auricle, Ear Canal, Surface of Ear Drum
The outer ear is the most external portion of the ear. The outer ear includes the pinna (also called auricle), the ear canal, and the very most superficial layer of the eardrum (also called the tympanic membrane). Although the word “ear” may properly refer to the pinna (the flesh covered cartilage appendage on either side of the head), this portion of the ear is not vital for hearing. The complicated design of the human outer ear does help capture sound, but the most important functional aspect of the human outer ear is the ear canal itself. This outer ear canal skin is applied to cartilage; the thinner skin of the deep canal lies on the bone of the skull. If the ear canal is not open, hearing will be dampened. Ear wax (medical name: cerumen) is produced by glands in the skin of the outer portion of the ear canal. Only the thicker cerumen-producing ear canal skin has hairs. The outer ear ends at the most superficial layer of the tympanic membrane. The tympanic membrane is commonly called the eardrum.
Middle Ear: Air Filled Cavity behind the Ear Drum, includes most of the Ear Drum, and Ear Bones
The middle ear includes most of the ear drum (tympanic membrane) and the 3 ear bones ossicles: malleus (or hammer), incus (or anvil), and stapes (or stirrup). The opening of the Eustachian tube is also within the middle ear. The malleus has a long process (the handle) that is attached to the mobile portion of the ear drum. The incus is the bridge between the malleus and stapes. The stapes is the smallest named bone in the human body. The stapes transfers the vibrations of the incus to the oval window, a portion of the inner ear to which it is connected. It is the final bone in the chain to transfer vibrations from the eardrum to the inner ear. The arrangement of these 3 bones is a sort of Rube Goldberg device: movement of the tympanic membrane causes movement of the first bone, which causes movement of the second, which causes movement of the third. When this third bone pushes down, it causes movement of fluid within the cochlea (a portion of the inner ear). This particular fluid only moves when the stapes footplate is depressed into the inner ear. Unlike the open ear canal, however, the air of the middle ear is not in direct contact with the atmosphere outside the body. The Eustachian tube connects from the chamber of the middle ear to the back of the pharynx. The middle ear in humans is very much like a specialized paranasal sinus, called the tympanic cavity, it, like the paranasal sinuses, is a hollow mucosa lined cavity in the skull that is ventilated through the nose. The mastoid portion of the temporal bone, which can be felt as a bump in the skull behind the pinna, also contains air, which ventilates through the middle ear.
Inner Ear: Cochlea, Vestibule, and Semi-Circular Canals
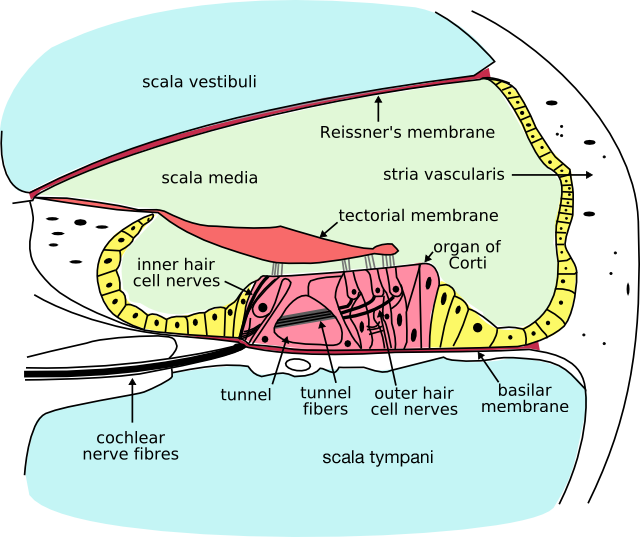
Figure 4. Cross section of the cochlea
The inner ear includes both the organ of hearing (the cochlea, Figure 4) and a sense organ (the labyrinth or vestibular apparatus) that is attuned to the effects of both gravity and motion. The balance portion of the inner ear consists of three semi-circular canals and the vestibule. The inner ear is encased in the hardest bone of the body. Within this ivory hard bone, there are fluid-filled hollows. Within the cochlea are three fluid filled spaces: the tympanic canal, the vestibular canal, and the middle canal. The eighth cranial nerve comes from the brain stem to enter the inner ear. When sound strikes the ear drum, the movement is transferred to the footplate of the stapes, which attaches to the oval window and presses into one of the fluid-filled ducts of the cochlea. The hair cells in the organ of Corti are stimulated by particular frequencies of sound, based on their location within the cochlea. High pitch sounds are at a higher frequency and, due to the shorter wavelength they “hit” the membrane “faster” (ie. close to the oval window). In contrast, low frequency sounds have large wavelengths, and will travel further through the scala vestibuli before “hitting” the tectorial membrane near the apex of the cochlea. The fluid inside the cochlea is moved, flowing against the receptor (hair) cells of the organ of Corti, which fire in a graded response based on the volume of the sound. The hair cells then stimulate the nerve cells in the Spiral Ganglion, which sends information through the auditory portion of the eighth cranial nerve to the brain. Humans are able to hear sounds between about 20 Hz and 20,000 Hz. Mammals that can hear lower frequency sounds, such as whales and elephants, have a longer cochlea. Humans tend to lose high-frequency hearing first, which has led some teenagers to using high-frequency ring tones (above 17,000 Hz) that may go undetected by their middle-aged teachers.
Hair Cell
Hair cells are columnar cells, each with a bundle of 100–200 specialized cilia at the top, for which they are named. These cilia are the mechanosensors for hearing. Lightly resting atop the longest cilia is the tectorial membrane, which moves back and forth with each cycle of sound, tilting the cilia and allowing electric current into the hair cell. Hair cells, like the photoreceptors of the eye, show a graded response, instead of the spikes typical of other neurons.
Immediately over the hair cells of the organ of Corti is an overhanging “tectorial membrane.” When the Bones of the Middle Ear vibrate the oval window, these vibrations are transmitted to the fluid within the cochlea and eventually cause the round window on the cochlea to bulge outward. These vibrations deflect the membrane on which the Organ of Corti is located, causing the three rows of outer hair cells to “rub” against the overhanging tectorial membrane. By their muscle-like activity they ampify the weakest vibrations for the inner hair cells. The louder sounds are not amplified. The disturbed inner hair cells will then activate the cochlear nerve fibers.
The current model is that cilia are attached to one another by “tip links,” structures which link the tips of one cilium to another. Stretching and compressing the tip links may open an ion channel and produce the receptor potential in the hair cell. These graded potentials are not bound by the “all or none” properties of an action potential. There are far fewer hair cells than afferent (leading to the brain) nerve fibers in the cochlea. The nerve that innervates the cochlea is the cochlear nerve, and forms cranial nerve number VIII with the vestibular nerve from the balance organ. Neuronal dendrites innervate cochlear hair cells. The neurotransmitter itself is thought to be glutamate. At the presynaptic juncture, there is a distinct “presynaptic dense body” or ribbon. This dense body is surrounded by synaptic vesicles and is thought to aid in the fast release of neurotransmitter. Efferent projections from the brain to the cochlea also play a role in the perception of sound. Efferent synapses occur on outer hair cells and on afferent dendrites under inner hair cells.
Process of Hearing
Detection of sound motion is associated with the right posterior superior temporal gyrus. The superior temporal gyrus contains several important structures of the brain, including: marking the location of the primary auditory cortex, the cortical region responsible for the sensation of sound. Sections 41 and 42 are called the primary auditory area of the cerebrum, and processes the basic characteristics of sound such as pitch and rhythm. The auditory association area is located within the temporal lobe of the brain, in an area called the Wernicke’s area, or area 22. This area, near the lateral cerebral sulcus, is an important region for the processing of acoustic energy so that it can be distinguished as speech, music, or noise. It also interprets words that are heard into an associated thought pattern of understanding. The gnostic area of the cerebrum, (areas 5, 7, 39 and 40) helps to integrate all incoming sense patterns so that a common thought can be formed (correlated) using all arriving sensory information.
Hearing Under Water
Hearing threshold and the ability to localize sound sources are reduced underwater, in which the speed of sound is faster than in air. Underwater, hearing is by bone conduction and localization of sound appears to depend on differences in amplitude detected by bone conduction.
Localization of Sound by Humans
Humans are normally able to hear a variety of sound frequencies, from about 20Hz to 20kHz. Our ability to estimate just where the sound is coming from, sound localization, is dependent on both hearing ability of each of the two ears, and the exact quality of the sound. Since each ear lies on an opposite side of the head, a sound will reach the closest ear first, and its amplitide will be loudest in that ear. Much of the brain’s ability to localize sound depends on interaural (between ears) intensity differences and interaural temporal or phase differences.
Two mechanisms are known to be used. Bushy neurons can resolve time differences as small as the time it takes sound to pass one ear and reach the other (10 milliseconds). For high frequencies, frequencies with a wavelength shorter than the listener’s head, more sound reaches the nearer ear. Human echolocation is a technique involving echolocation used by some blind humans to navigate within their environment.
Process of Equilibrium
Equilibrioception or sense of balance is one of the physiological senses. It allows humans and animals to walk without falling. Some animals are better in this than humans, for example allowing a cat (as a quadruped using its inner ear and tail) to walk on a thin fence. All forms of equilibrioception can be described as the detection of acceleration.
It is determined by the level of fluid properly called endolymph in the labyrinth: a complex set of tubing in the inner ear.
When the sense of balance is interrupted it causes dizziness, disorientation and nausea.
You can temporarily disturb your sense of balance by closing your eyes and turning rapidly in circles five or six times. This starts the fluid swirling in circles inside your ear canal. When you stop turning it takes a few seconds for the fluid to lose momentum, and until then the sense from your inner ear conflicts with the information coming from your vision, causing dizziness and disorientation. Most astronauts find that their sense of balance is impaired when in orbit, because there is not enough gravity to keep the ear’s fluid in balance. This causes a form of motion sickness called space sickness.
Touch
Touch is the first sense developed in the womb and the last sense used before death. With 50 touch receptors for every square centimeter and about 5 million sensory cells overall, the skin is very sensitive and is the largest and one of the most complex organs in our bodies. These touch receptors are grouped by type and include mechanoreceptors (sensitive to pressure, vibration and slip), thermoreceptors (sensitive to changes in temperature), and nocioreceptors (responsible for pain).
Pacinian Corpuscles
Pacinian corpuscles detect gross pressure changes and vibrations. They are the largest of the receptors. Any deformation in the corpuscle causes action potentials to be generated, by opening pressure-sensitive sodium ion channels in the axon membrane. This allows sodium ions to influx in, creating a receptor potential. Pacinian corpuscles cause action potentials when the skin is rapidly indented but not when the pressure is steady, due to the layers of connective tissue that cover the nerve ending [3]. It is thought that they respond to high velocity changes in joint position.
Meissner’s Corpuscle
Meissner’s corpuscles are distributed throughout the skin, but concentrated in areas especially sensitive to light touch, such as the fingertips, palms, soles, lips, tongue, face, nipples and the external skin of the male and female genitals. They are primarily located just beneath the epidermis within the dermal papillae. Any physical deformation in the Meissner’s corpuscle will cause an action potential in the nerve. Since they are rapidly adapting or phasic, the action potentials generated quickly decrease and eventually cease. If the stimulus is removed, the corpuscle regains its shape and while doing so (i.e., while physically reforming) causes another volley of action potentials to be generated. This is the reason one stops “feeling” one’s clothes. This process is called sensory adaption. Because of their superficial location in the dermis, these corpuscles are particularly sensitive to touch and vibrations, but for the same reasons, they are limited in their detection because they can only signal that something is touching the skin. Meissner’s corpuscles do not detect pain; this is signaled exclusively by free nerve endings.
Ruffini Corpuscles
Ruffini corpuscles are thermoreceptors, aiding in the detection of temperature changes. Named after Angelo Ruffini, the Ruffini ending is a class of slowly adapting mechanoreceptor thought to exist only in the glabrous dermis and subcutaneous tissue of humans. This spindle-shaped receptor is sensitive to skin stretch, and contributes to the kinesthetic sense of and control of finger position and movement.
Try It
Candela Citations
- Human Physiology. Provided by: Wikibooks. Located at: https://en.wikibooks.org/wiki/Human_Physiology. License: CC BY-SA: Attribution-ShareAlike
- Turin L (1996). "A spectroscopic mechanism for primary olfactory reception." Chem. Senses. 21 (6): 773–91. ↵
- Keller A, Vosshall LB. "Olfactory perception of chemically diverse molecules." PubMed.gov. https://www.ncbi.nlm.nih.gov/pubmed/27502425. ↵
- Kandel ER, Schwartz JH, Jessell TM 2000. Principles of Neural Science, 4th ed. McGraw-Hill, New York. ↵